aFosphenytoin is a prodrug for phenytoin.
bOxcarbazepine serves largely as a prodrug for licarbazepine, mainly S-licarbazepine.
cEslicarbazepine acetate is a prodrug for S-licarbazepine.
dGabapentin enacarbil is a prodrug for gabapentin.
Many AED targets are ion channels, most notably voltage- gated sodium and potassium channels and GABAA receptors. It is interesting to note that certain idiopathic epilepsy syndromes are believed to be the result of mutations in these same ion channels (see Chapter 4).
VOLTAGE-GATED ION CHANNELS
Voltage-Gated Sodium Channels
Voltage-gated sodium channels play an essential role in the initiation and propagation of action potentials in neurons. Neuronal depolarizations by a few millivolts, ordinarily as a result of synaptic activation of glutamate receptors (mainly AMPA receptors), activate sodium channels, causing opening of the channels and influx of sodium. The channels then inactivate within milliseconds. Influx of sodium ions during the brief time that sodium channels are open generates the depolarizing component of the action potential. Although the bulk of sodium channels inactivate, about 1% of the sodium current is noninactivating resulting in a small persistent sodium current (INaP), which is carried by the same channels as the fast transient current. INaP facilitates epileptic burst firing by reducing the threshold for action potential generation, sustaining repetitive firing, and enhancing depolarizing synaptic currents (1). Some AEDs, most notably phenytoin, inhibit INaP, which is believed to contribute to their efficacy (2).
Voltage-gated sodium channels are multimeric protein complexes, composed of a large α subunit that forms four subunit-like homologous domains (designated I to IV) and one or more smaller β subunits (3). The ion-conducting pore is contained within the α subunit, as are the elements of the channel that mediate its fundamental physiologic properties including rapid inactivation. There are nine voltage-gated sodium channels, designated Nav1.1 to Nav1.9. Nav1.2 is the predominant form in brain neurons, but Nav1.1 and Nav1.6 are also expressed in the brain. Mutations in each of these channels have been associated with various genetic epilepsies (4).
AEDs that protect against seizures through an interaction with voltage-gated sodium channels are commonly referred to as “sodium channel blockers.” They are among the most frequently used drugs in the treatment of focal and primary generalized tonic–clonic seizures and include phenytoin, carbamazepine, lamotrigine, oxcarbazepine (as well as its active metabolite licarbazepine), and lacosamide. AEDs that interact with voltage-gated sodium channels exhibit a characteristic “use-dependent” blocking action so that they inhibit high-frequency trains of action potentials much more potently than they inhibit individual action potentials or firing at low frequencies. Because they also exhibit a “voltage dependence” to their blocking action, sodium channel–blocking AEDs are more potent at inhibiting action potentials that ride on a depolarized plateau potential as characteristically occurs in seizures. Thus, sodium channel–blocking AEDs preferentially inhibit seizure discharges in relation to normal ongoing neural activity. By virtue of their ability to inhibit the action potential invasion of nerve terminals, sodium channel–blocking AEDs inhibit the release of diverse neurotransmitters including glutamate; whether this is responsible for the therapeutic activity of the drugs is uncertain (5).
The binding site on sodium channels for sodium channel–blocking AEDs is believed to overlap the binding site of local anesthetics, which is within the pore of the channel and is formed by the S6 segments of domains I, II, and IV. Sodium channel–blocking AEDs bind with higher affinity to this site when the channel is in the inactivated state, and, when such a drug is bound, the channel is stabilized in the inactivated state. When neurons are depolarized and firing rapidly, sodium channels spend a greater amount time in the inactivated state and are able to accumulate bound drug so that they become trapped in the inactivated state. This accounts for the use- and voltage-dependent blocking action that they exhibit. Phenytoin, carbamazepine, and lamotrigine are considered “classical” sodium channel–blocking AEDs. Lacosamide also is believed to exert its therapeutic effects by interacting with sodium channels (6). Unlike other sodium channel–blocking AEDs, lacosamide does not inhibit high-frequency repetitive spike firing on the time scale of 100s of milliseconds. It does, however, inhibit spike firing in long trains of spikes on the time scale of 1 to 2 seconds. It has been proposed that the very slow action of lacosamide is due to an enhancement of a distinct and poorly understood form of inactivation, referred to as “slow inactivation.” However, an alternative explanation is that lacosamide binds more slowly to fast inactivated sodium channels than do the other sodium channel–blocking AEDs. In any case, the unusually slow development of block produced by lacosamide during high-frequency activity could allow lacosamide to better discriminate between seizure-like pathologic firing and normal network activity.
T-Type Voltage-Gated Calcium Channels
Low voltage-activated (T-type) calcium channels play a role in the intrinsic thalamocortical oscillations that underlie the spike-and-wave discharges of generalized absence seizures (7–9). There are three T-type Ca2+ channel isoforms encoded by separate genes, denoted as Cav3.1 (α1G), Cav3.2 (α1H), and Cav3.3 (α1I). All three T-type calcium channel isoforms are expressed in thalamocortical circuits (10). Cav3.1 is prominently expressed in thalamic relay neurons in the dorsal thalamus, which plays a key role in absence seizures; Cav3.2 and to a lesser extent Cav3.3 are prominently expressed in thalamic reticular neurons. All three T-type calcium channel isoforms are expressed in the cortex, with Cav3.2 mainly localized to layer V. In non-REM sleep, including during delta waves, sleep spindles, and K complexes, the thalamocortical circuit switches from a tonic to oscillatory mode of firing, but in absence epilepsy, this switching can occur inappropriately, even during wakefulness (11,12). T-type calcium channels in the thalamus and cortex contribute to the abnormal behavior of the circuit. These channels generate low-threshold spikes, leading to burst firing and oscillatory behavior (13). GABA-ergic neurons of the thalamic reticular nucleus are also critically involved in absence seizures as they hyperpolarize thalamic relay neurons, which deinactivate T-type calcium channels allowing the channels to generate burst firing and the propagation of spike-and-wave discharges in the thalamocortical circuit (14).
Ethosuximide, which is highly efficacious in the treatment of absence seizures but not other seizure types, seems to act by inhibition of T-type calcium channels in the thalamocortical circuit (15–17). At clinically relevant concentrations (20 to 40 μg/mL), some but not all investigators have observed a partial (20% to 30%) reduction of T-type calcium current by ethosuximide. However, studies with recombinant T-type calcium channels have confirmed that ethosuximide blocks all three channel types (18). The block increases when the current is activated from more depolarized potentials and when T-type calcium channels are inactivated as especially occurs during high-frequency activation, so that the drug has selectivity for pathologic behavior in the thalamocortical circuit, which is associated with neuronal depolarization and inactivation of T-type calcium channels. Effects on other membrane currents, including INaP and calcium-activated potassium current, may contribute to the efficacy of ethosuximide in absence epilepsy (17). Remarkably, results in animal models indicate that early treatment with ethosuximide can have disease-modifying (antiepileptogenic) effects, causing a persistent reduction in seizures and mitigation of behavioral comorbidities (19,20). These actions may be caused by epigenetic modifications. A study showing that children with absence epilepsy who receive ethosuximide are more likely than those who receive valproic acid to achieve long-term remission is consistent with the disease-modifying actions observed in animal studies (21).
The efficacy of some other AEDs may also depend, at least in part, on actions at T-type calcium channels. Zonisamide, in addition to effects on voltage-activated sodium channels, may also block T-type calcium channels (11), thus accounting for its likely efficacy in absence epilepsy (22). Similarly, there is evidence that valproate, a drug of choice in absence epilepsy, may also inhibit T-type calcium channels (17).
Kv7 Voltage-Gated Potassium Channels
Voltage-gated potassium channels are a diverse and evolutionarily ancient group of ion channels that serve a variety of key functions in the nervous system. Opening of potassium channels drives the membrane potential toward a hyperpolarized level, which serves to repolarize depolarizing events (such as action potentials and synaptic potentials) and cause a generalized reduction in excitability. In 1998, the first genes for a human idiopathic epilepsy were identified (23). These genes, designated KCNQ2 and KCNQ3, encoded novel brain potassium channel subunits, Kv7.2 and Kv7.3, respectively, that are homologous to a previously identified cardiac potassium channel Kv7.1, encoded by KCNQ1 (LQT1). The novel brain potassium channels mediate the M-current, a potassium current that increases as the membrane potential in neurons approach action potential threshold. Kv7 channels, together with HCN (hyperpolarization-activated cyclic nucleotide-gated potassium channels) and KCa2/SK (small-conductance calcium-activated potassium channels), generate the medium after hyperpolarization, which is elicited by a burst of action potentials and serves to limit further firing (24). Kv7 potassium channels therefore contribute to spike- frequency adaptation and can be considered to serve as a “brake” on epileptic firing. The Kv7 family of potassium channels is now known to contain five members, including Kv7.1, which is expressed predominantly in the heart, and Kv7.2 to Kv7.5, which are expressed exclusively in the nervous system (25).
Ezogabine, which is efficacious in the treatment of partial seizures, acts as a positive modulator of the nervous system Kv7 potassium channels (Kv7.2 to Kv7.5) but does not affect the cardiac member of the family (Kv7.1). Of particular relevance to the antiseizure action of ezogabine is its action on the M-current, which is predominantly carried by channels composed of Kv7.2 and Kv7.3, although Kv7.5 alone or in combination with Kv7.3 also contributes (26,27). Ezogabine causes a hyperpolarizing shift in the activation of Kv7 channels such that more M-current is generated near resting potential. It also causes a change in the kinetics of single KCNQ channels to favor channel opening, thus increasing the macroscopic M-current; ezogabine does not alter the single-channel conductance of individual Kv7 channels (28). Many Kv7 channels in the brain are believed to be Kv7.2/Kv7.3 heteromers, which are highly sensitive to ezogabine (EC50, 1.6 μM) (27). Peak plasma levels of ezogabine range from 354 to 717 ng/mL (1.2 to 2.4 μM) (29), and plasma protein binding is 80% so that free plasma concentrations are estimated to be about 0.2 to 0.5 μM; brain concentrations are expected to be similar. Therefore, therapeutic concentrations likely only modestly potentiate the most sensitive Kv7 channels and do not affect less sensitive channels. The binding site for ezogabine in Kv7.2/Kv7.3 heteromers is in a pocket formed by the pore-lining S5 membrane segment of one subunit and the pore-lining S6 membrane segment of the neighboring subunit (30,31). Channel opening may expose the pocket, permitting binding of ezogabine, which stabilizes the open-channel conformation.
GABA INHIBITION
GABA, the neurotransmitter of local inhibitory interneurons, acts through GABAA receptors and GABAB receptors. GABAA receptors, which are Cys loop-type ligand-gated chloride channels, represent an important target for AEDs and will be considered here; GABAB receptors, which are heterodimeric G protein–coupled receptors that activate potassium channels and inhibit calcium channels, are distinct in structure and function from GABAA receptors and are not a target of any AED. Although only about one in five cortical neurons is GABA-ergic (32), these neurons play a critical role in controlling the firing rate and timing of principal (excitatory) neurons. In addition, they synchronize local neuronal ensembles and restrain the generation of abnormal epileptic behavior. Consequently, enhancement of GABA-ergic inhibition is a key mechanism of AED action.
GABAA Receptors
GABAA receptors are heteropentameric protein complexes localized to the postsynaptic membrane of inhibitory synapses where they mediate fast neuronal inhibition on a millisecond time scale. They are also located extrasynaptically where they respond to ambient GABA in the extracellular milieu and confer tonic (long-term) inhibition. There are 19 known GABAA receptor subunits (α1 to 6, β1 to 3, γ1 to 3, δ, ε, θ, π, and ρ1 to 3). However, the bulk (60%) of synaptic GABAA receptors are believed to have the α1β2γ2 configuration, and a considerable fraction of the remainder (15% to 20%) are α2β3γ2. Among the receptor subtypes that contribute to tonic signaling in the brain regions relevant to epilepsy are α4βxδ receptors, which are believed to mediate the tonic current in dentate granule cells and thalamocortical neurons, and α5-containing GABAA receptors in CA1 pyramidal cells (33).
Benzodiazepines, such a diazepam, lorazepam, and clonazepam, and barbiturates, such as phenobarbital, are AEDs that act on GABAA receptors as positive allosteric modulators. At higher concentration, barbiturates can directly activate GABAA receptors in the absence of GABA (34), whereas benzodiazepines cannot. Benzodiazepines are specific for synaptic GABAA receptors containing the γ2 subunit and act to allosterically modulate these receptors to increase the channel opening frequency resulting in enhanced synaptic inhibition. This confers a broad-spectrum anticonvulsant action. In most epilepsy syndromes, the specific cellular types that are involved in the antiseizure activity of benzodiazepines are not known. However, in the case of absence epilepsy, it is believed that benzodiazepines desynchronize the thalamocortical oscillations underlying generalized spike-and-wave discharges by specific effects on α3-containing GABAA receptors in the thalamic reticular nucleus (35). Barbiturates, presumably because they are not specific for α3-containing GABAA receptors, are not active in absence epilepsy and may even aggravate absence seizures. In contrast to benzodiazepines, barbiturates do not appear to increase the frequency of GABA-induced chloride channel opening, but instead increase the channel open time. In addition to effects on GABAA receptors, barbiturates modulate other ion channel systems, including calcium and sodium channels, and these actions may contribute to therapeutic activity (36).
GAT-1 GABA Transporter
The action of neurotransmitter GABA is terminated by uptake into neurons and glial cell by membrane-bound GABA transporters, of which there are four types, termed GAT-1, BGT-1, GAT-2, and GAT-3. GAT-1 (encoded by the SLC6A1 gene), the predominant form in the forebrain (including the neocortex and hippocampus), is localized to GABA-ergic terminals as well as to glial processes near GABA synapses. Tiagabine is a highly selective inhibitor of GAT-1 in neurons and glia (37). Inhibition of GAT-1 by tiagabine suppresses the translocation of extracellular GABA into the intracellular compartment, thus raising extracellular GABA levels. Functionally, tiagabine prolongs GABA-mediated inhibitory synaptic responses, and the marked elevation in extracellular GABA it produces may lead to activation of extrasynaptic GABA receptors.
GABA Transaminase
4-Aminobutyrate aminotransferase (GABA transaminase), an enzyme that catalyzes the conversion of GABA and 2-oxoglutarate into succinic semialdehyde and glutamate, is responsible for the metabolic inactivation of GABA. Inhibition of GABA transaminase with vigabatrin (γ-vinyl GABA), an irreversible suicide inhibitor of the enzyme, leads to marked increases in brain GABA levels. Although the antiseizure action of vigabatrin is believed to reflect inactivation of GABA transaminase, how this occurs is not straightforward and does not appear to be due to an enhancement of inhibitory synaptic transmission. In contrast to the action of tiagabine, vigabatrin does not elicit larger or more prolonged GABAA receptor–mediated synaptic responses (38,39). Rather, preincubation of brain slices with vigabatrin irreversibly inhibited miniature and evoked inhibitory postsynaptic currents. Additional experiments suggested that the paradoxical effect resulted from a reduction in the GABA content of synaptic vesicles caused by GABA transaminase inhibition. In contrast to the effect on GABA-mediated synaptic transmission, vigabatrin caused an increase in nonsynaptic tonic GABAA receptor current. This steady current is believed to be mediated by the action of GABA in the extracellular milieu acting on extrasynaptic GABAA receptors. High levels of intracellular GABA cause a reversal of GABA transporters, resulting in a marked elevation in extracellular GABA, which is likely responsible for the increase in tonic GABAA receptor current. It can be concluded that vigabatrin causes divergent effects on synaptic and extrasynaptic GABA-mediated inhibition, with seizure protection resulting from a predominance of the extrasynaptic action. Interestingly, in the early period after administration of vigabatrin to animals, there is a reduction in seizure threshold, whereas the anticonvulsant actions become evident only later (40,41). Thus, vigabatrin has a biphasic action with proconvulsant effects likely related to suppression of synaptic GABA-ergic neurotransmission and anticonvulsant effects due to spillover of GABA into the extracellular space and activation of extrasynaptic GABAA receptors. Interestingly, individuals with a rare genetic deficiency of GABA transaminase experience refractory seizures, supporting the view that inhibition of GABA transaminase is in fact the proconvulsant mechanism of vigabatrin (42).
SYNAPTIC RELEASE MACHINERY
SV2A
A variety of lines of evidence support the conclusion that SV2A, a membrane glycoprotein found in the secretory vesicles of neurons and endocrine cells and possibly immune cells, is the molecular target for levetiracetam (43,44). There is a strong correlation between the affinity of levetiracetam analogs for binding to SV2A and the potency of the analogs in several animal seizure models. Moreover, seizure protection conferred by levetiracetam and other SV2A ligands strongly correlates with the degree of SV2A occupancy in vivo. Finally, the anticonvulsant efficacy of levetiracetam but not valproate, which does not interact with SV2A, is reduced in SV2A+/− mice that have one copy of SV2A disrupted by gene targeting. The precise way in which binding of levetiracetam to SV2A leads to seizure protection is not understood.
Indeed, the function of SV2A itself is obscure. Among the various functions proposed are roles in calcium-dependent exocytosis, neurotransmitter loading/retention in synaptic vesicles, and synaptic vesicle priming, as well as transport of vesicle constituents. SV2A is one of three homologous of SV2 proteins that belong to the major facilitator superfamily of 12-transmembrane domain transporters. Despite substantial effort, no transport function of these proteins has been identified, although studies with protein tomography have found that SV2A can adopt two alternate conformations consistent with a transporter role (45). Interestingly, however, levetiracetam binding does not cause a large-scale conformational change in SV2A or lock a specific conformational state of the protein as would an inhibitor of transport. Apparently, the drug has a more subtle effect on the protein. Although the function of SV2A is still poorly defined, SV2A−/− knockout mice exhibit a lethal seizure phenotype demonstrating that SV2A in some way serves to restrain seizures.
A series of recent studies has examined the impact of levetiracetam on synaptic transmission in brain slice recordings (46). Although the drug had no effect on synaptic physiology with low-frequency activation, levetiracetam did reduce the synaptic release of both excitatory (glutamate) and inhibitory (GABA) neurotransmitters during high-frequency activation. The frequency dependence is compatible with the selective suppression of epileptic activity. Modulation of synaptic release is a common mechanism of many AEDs, including sodium channel blockers that indirectly inhibit release at both excitatory and inhibitory synapses by inhibiting action potential firing. It seems that drugs that suppress inhibition and excitation can effectively protect against seizures and they are not often proconvulsant. However, it is noteworthy that in some instances AEDs (notably phenytoin) can have proconvulsant effects.
α2δ-1
The gabapentinoids gabapentin and pregabalin act by binding to the α2δ-1 protein, which is an accessory subunit of voltage-gated calcium channels (47,48). α2δ-1 is located heterogeneously in the brain, particularly at presynaptic sites on excitatory (glutamatergic) neurons. Dense expression is observed in areas relevant to epilepsy, including in excitatory hippocampal mossy fibers and in the neocortex and amygdala. In contrast, α2δ-1 has minimal expression in the thalamus, and it is noteworthy that gabapentinoids are not active in absence seizures, which as discussed above are dependent upon this brain structure. Four α2δ subunits have been identified, but gabapentinoids only bind to α2δ-1 and α2δ-2 owing to the presence of an RRR motif containing a critical arginine that is required for binding. Seizure protection conferred by gabapentinoids is eliminated in mice bearing a mutation in this motif (RRR mutated to RRA) in α2δ-1, demonstrating that α2δ-1 and not α2δ-2 is relevant for pharmacologic activity. Interestingly, deletion of α2δ-1 or α2δ-2 in mice is associated with absence epilepsy or enhanced seizure susceptibility (49,50).
The precise way in which binding of gabapentin and pregabalin to the α2δ-1 protein confers seizure protection is not well understood (51). Although some studies have found that the drugs inhibit calcium channel currents, most have not and it is generally believed that calcium channel inhibition is not the mechanism of action of gabapentinoids (52–54). Regardless of whether the drugs inhibit calcium channel function, they do seem to block the release of various neurotransmitters, including glutamate, and this may account for the antiseizure activity (55). There is some evidence that gabapentinoids cause internalization of calcium channels by reducing trafficking to the cell membrane (56,57
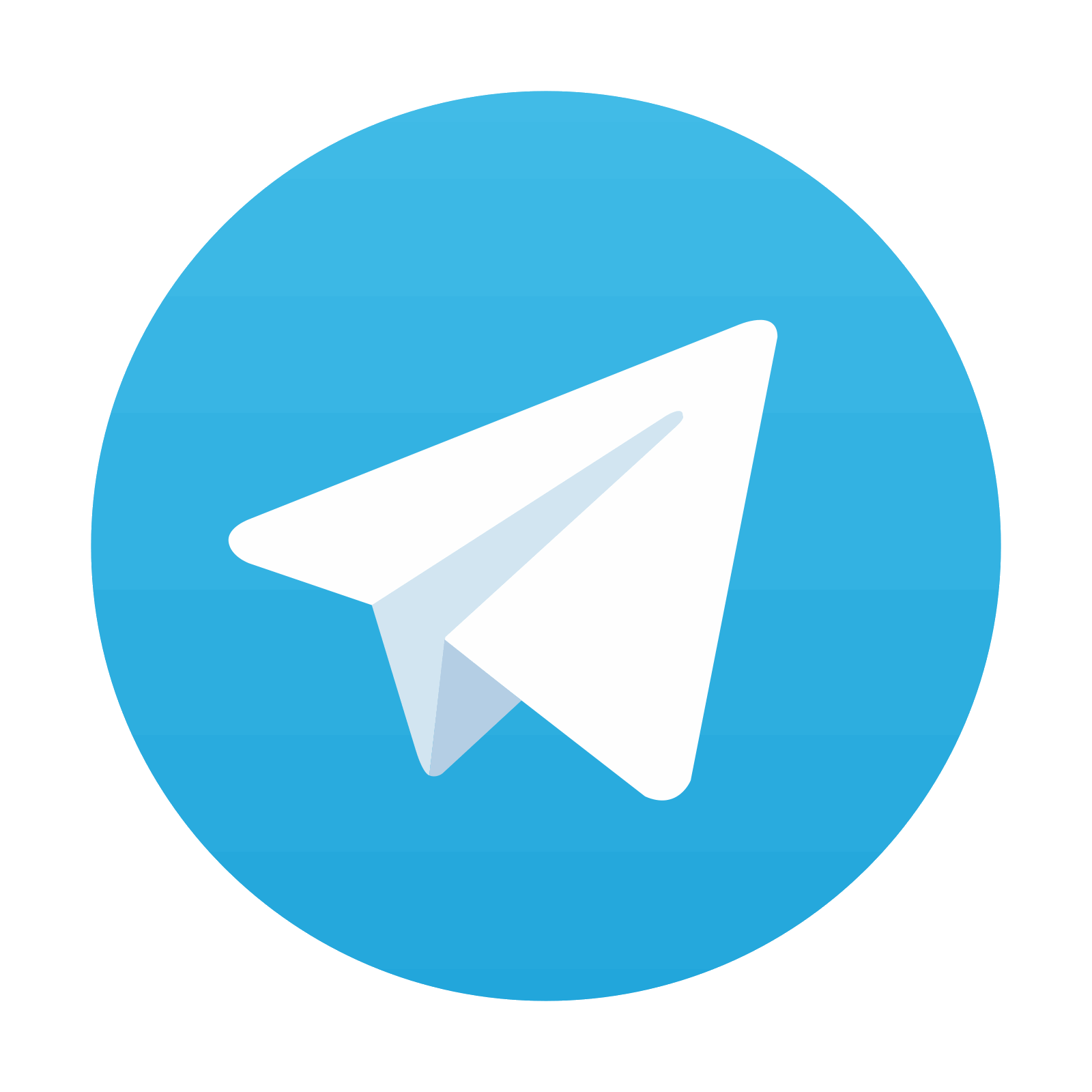
Stay updated, free articles. Join our Telegram channel
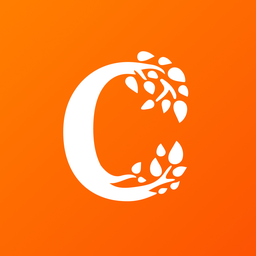
Full access? Get Clinical Tree
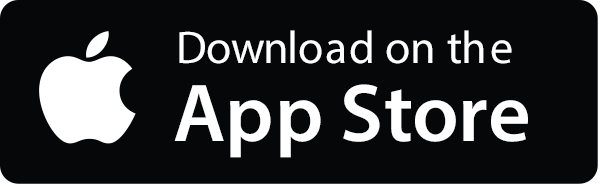
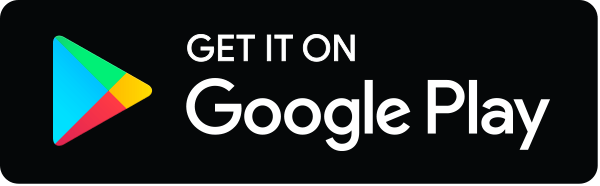