Modulation of Synaptic Transmission: Second Messengers
A Family of G Proteins Activates Distinct Second-Messenger Pathways
Transcellular Messengers Are Important for Regulating Presynaptic Function
The Gaseous Second Messengers, Nitric Oxide and Carbon Monoxide, Stimulate Cyclic GMP Synthesis
A Family of Receptor Tyrosine Kinases Mediates Some Metabotropic Receptor Effects
The Physiological Actions of Ionotropic and Metabotropic Receptors Differ
Second-Messenger Cascades Can Increase or Decrease the Opening of Many Types of Ion Channels
Cyclic AMP-Dependent Protein Phosphorylation Can Close Potassium Channels
Synaptic Actions Mediated by Phosphorylation Are Terminated by Phosphoprotein Phosphatases
Second Messengers Can Endow Synaptic Transmission with Long-Lasting Consequences
THE BINDING OF NEUROTRANSMITTER to postsynaptic receptors produces a postsynaptic potential either directly, by opening ion channels, or indirectly, by altering ion channel activity through changes in the postsynaptic cell’s biochemical state. As we saw in Chapter 8, the type of action depends on the type of receptor. Activation of ionotropic receptors directly opens ion channels that are part of the receptor macro-molecule itself. In contrast, activation of metabotropic receptors regulates the opening of ion channels indirectly through biochemical signaling pathways. The receptor and ion channels that are affected are distinct macromolecules (Figure 11-1).
Figure 11-1 Neurotransmitter actions can be divided into two groups according to the way in which receptor and effector functions are coupled.
A. Direct transmitter actions are produced by ionotropic receptors, ligand-gated channels in which the receptor and ion channel are domains formed by a single macromolecule. The binding of transmitter to the receptor on the extracellular aspect of the protein directly opens the ion channel embedded in the cell membrane.
B. Indirect transmitter actions are caused by binding of transmitter to metabotropic receptors that are separate macromolecules from the ion channels that they regulate. There are two families of these receptors. 1. G protein-coupled receptors activate guanosine triphosphate (GTP)-binding proteins that engage a second-messenger cascade or act directly on ion channels. 2. Receptor tyrosine kinases initiate a cascade of protein phosphorylation reactions, beginning with autophosphorylation of the kinase itself on tyrosine residues.
Whereas the action of ionotropic receptors is fast and brief, metabotropic receptors produce effects that begin slowly and persist for long periods, ranging from hundreds of milliseconds to many minutes. The two types of receptors also differ in their functions. Ionotropic receptors mediate behaviors, from simple reflexes to complex cognitive processes. Metabotropic receptors modulate behaviors; they modify reflex strength, help focus attention, set emotional states, and contribute to long-lasting changes in neural circuits that underlie learning and memory. Metabotropic receptors are responsible for many of the actions of transmitters, hormones, and growth factors.
Ionotropic receptors change the balance of charge across the neuron’s membrane quickly. As we have seen, this change is local at first but is propagated as an action potential along the axon if the change in membrane potential is suprathreshold. Activation of metabotropic receptors also begins as a local action that can spread to a wider region of the cell. A neurotransmitter reacting with a metabotropic receptor activates proteins that in turn activate effector enzymes. The effector enzymes then often produce second-messenger molecules that can diffuse within a cell to activate still other enzymes that catalyze modifications of a variety of target proteins, greatly changing their activities.
There are two major families of metabotropic receptors: G protein-coupled receptors and receptor tyrosine kinases. We first describe the G protein-coupled receptor family and later discuss the receptor tyrosine kinase family.
The G protein-coupled receptors are coupled to an effector by a trimeric guanine nucleotide-binding protein, or G protein (Figure 11-1B). This receptor family contains α- and β-adrenergic receptors for nore-pinephrine, muscarinic acetylcholine (ACh) receptors, γ-aminobutyric acid B (GABAB) receptors, certain glutamate and serotonin receptors, all receptors for dopamine, receptors for neuropeptides, odorant receptors, rhodopsin (the protein that reacts to light, initiating visual signals, see Chapter 26), and many others. Many of these receptors are thought to be involved in neurological and psychiatric disease and are key targets for the actions of important classes of therapeutic drugs.
G protein-coupled receptors activate a variety of effectors. Typically the effector is an enzyme that produces a diffusible second messenger. These second messengers in turn trigger a biochemical cascade, either by activating specific protein kinases that phos-phorylate the hydroxyl group of specific serine or threonine residues in various proteins or by mobilizing Ca2+ ions from intracellular stores, thus initiating reactions that change the cell’s biochemical state. In some instances the G protein or the second messenger act directly on an ion channel.
The Cyclic AMP Pathway Is the Best Understood Second-Messenger Signaling Cascade Initiated by G Protein-Coupled Receptors
The adenosine 3′,5′-cyclic monophosphate (cyclic AMP or cAMP) pathway is a prototypic example of a second-messenger cascade. It was the first second-messenger pathway to be discovered, and our conception of other second-messenger pathways is based on it.
The binding of transmitter to receptors linked to the cAMP cascade first activates a specific G protein, Gs (named for its action to stimulate cAMP synthesis). In its resting state Gs′ like all G proteins, binds a molecule of guanosine diphosphate (GDP). The interaction of Gs with a ligand-bound receptor promotes the exchange of the bound GDP for guanosine triphosphate (GTP), leading to a conformational change that activates the G protein. In its activated state Gs stimulates the integral membrane protein adenylyl cyclase to catalyze the conversion of adenosine triphosphate (ATP) to cAMP. When associated with the cyclase, Gs also acts as a GTPase, hydrolyzing its bound GTP to GDP. When GTP is hydrolyzed, the G protein becomes inactive and dissociates from the cyclase, thereby stopping the synthesis of cAMP (Figure 11–2). Typically, a Gs protein remains active for a few seconds before its bound GTP is hydrolyzed.
Figure 11-2 The cAMP cycle. (Opposite) The binding of a transmitter to certain metabotropic receptors activates the stimulatory G protein (Gs). The G protein is a heterotrimer consisting of α-, β-, and γ-subunits. The β- and γ-subunits form a unit that binds tightly to the membrane. In its resting state the α-subunit of Gs, termed αs′, binds a molecule of guanosine diphosphate (GDP). When activated by its interaction with a ligand-bound receptor, the GDP bound to αs is exchanged for guanosine triphosphate (GTP), causing αs to functionally dissociate from the βγ complex. Next αs associates with an intracellular domain of adenylyl cyclase, thereby activating the enzyme to produce cAMP from adenosine triphosphate (ATP). When bound to the cyclase, αs is a GTPase. The hydrolysis of GTP to GDP and inorganic phosphate (Pi) leads to the dissociation of αs from the cyclase and its reassociation with the βγ complex. The cyclase then stops producing the second messenger. At some point during this cycle the transmitter dissociates from the receptor. The system returns to an inactive state when the transmitter-binding site on the receptor is empty, the three subunits of the G protein reassociate, and the guanine nucleotide-binding site on the a-subunit is occupied by GDP (Adapted, with permission, from Alberts et al. 1994.)
Once a G protein-coupled receptor binds a ligand, it can interact sequentially with more than one G protein macromolecule. As a result, the sequential binding of relatively few molecules of transmitter to a small number of receptors can activate a large number of cyclase complexes. The signal is further amplified in the next step in the cAMP cascade, the activation of the protein kinase.
The major target of cAMP in most cells is the cAMP-dependent protein kinase (also called protein kinase A or PKA). This kinase, identified and characterized by Edward Krebs and colleagues, is a het-erotetrameric enzyme consisting of a dimer of two regulatory (R) subunits and two catalytic (C) subunits. In the absence of cAMP the R subunits bind to and inhibit the C subunits. In the presence of cAMP each R subunit binds two molecules of cAMP, leading to a conformational change that causes the R and C sub-units to dissociate (Figure 11–3). Dissociation frees the C subunits to transfer the γ-phosphoryl group of ATP to the hydroxyl groups of specific serine and threonine residues in substrate proteins.
Figure 11-3 The cAMP pathway activates protein kinase A. Adenylyl cyclase converts adenosine triphosphate (ATP) into cAMP. Four cAMP molecules bind to the two regulatory sub-units of the protein kinase A (PKA), liberating the two catalytic subunits, which are then free to phosphorylate specific substrate proteins on certain serine or threonine residues, thereby producing a cellular response. Two kinds of enzymes regulate this pathway. Phosphodiesterases convert cAMP to adenosine monophosphate (which is inactive), and protein phosphatases remove phosphate groups (P) from the substrate proteins, releasing inorganic phosphate, Pi (see also Figure 11-14).
Protein kinase A is distantly related through evolution to other serine and threonine protein kinases that we shall consider: the Ca2+/calmodulin-dependent protein kinases and protein kinase C. These kinases also have regulatory and catalytic domains, but both domains are within the same polypeptide molecule (see Figure 11-6).
In addition to blocking enzymatic activity, the R subunits of PKA also target the C subunits to distinct sites within cells. Human PKA has two types of R sub-units, each with two subtypes: RIα, RIβ, RIIα, and RIIβ. The genes for each are distinct but derive from a common ancestor. The two R subunits in a molecule of PKA are present as a dimer, formed when the R subunits are synthesized. Because the dimer never separates, hybrid types of the kinase do not exist in the cell. This is functionally important because the types have different properties. For example, type II PKA (containing RII-type subunits) is targeted to the membrane by A kinase attachment proteins (AKAPs). One AKAP targets PKA to the N-methyl-D-aspartate (NMDA)-type glutamate receptor by binding both PKA and the postsynaptic density protein PSD-95, which binds to the cytoplasmic tail of the NMDA receptor (see Chapter 10). In addition, this AKAP also binds a protein phos-phatase, which removes the phosphate group from substrate proteins (see Figure 11-14). By localizing PKA and other signaling components near their substrate, AKAPs form local signaling complexes that increase the specificity, speed, and efficiency of second-messenger cascades. Because AKAPs have only a weak affinity for RI subunits, most type I PKA is free in the cytoplasm.
Kinases can only phosphorylate proteins on serine and threonine residues that are embedded within a context of specific phosphorylation consensus sequences of amino acids. For example, phosphorylation by PKA usually requires a sequence of two contiguous basic amino acids—either lysine or arginine—followed by any amino acid, and then by the serine or threonine residue that is phosphorylated (for example, Arg-Arg-Ala-Thr).
Several important protein substrates for PKA have been identified in neurons. These include voltage-gated and ligand-gated ion channels, synaptic vesicle proteins, enzymes involved in transmitter biosynthesis, and proteins that regulate gene transcription. As a result, the cAMP pathway has widespread effects on the electro-physiological and biochemical properties of neurons. We shall consider some of these actions later in this chapter.
The Second-Messenger Pathways Initiated by G Protein-Coupled Receptors Share a Common Molecular Logic
Approximately 800 of the roughly 23,000 genes thought to comprise the human genome code for G protein-coupled receptors. Although many of these are odorant receptors in olfactory neurons (see Chapter 32), many others are receptors for well-characterized neurotrans-mitters used throughout the nervous system. Despite their enormous diversity, all G protein-coupled receptors consist of a single polypeptide with seven characteristic membrane-spanning regions (serpentine receptors) (Figure 11–4).
Figure 11-4 G protein-coupled receptors contain seven membrane-spanning domains. The β2-adrenergin receptor shown here is representative of G protein-coupled receptors, including the β1-adrenergic and muscarinic acetylcholine (ACh) receptors and rhodopsin. It consists of a single subunit with an extracellular amino terminus, intracellular carboxy terminus, and seven membrane-spanning α-helixes. The binding site for the neurotransmitter lies in a cleft in the receptor formed by the transmembrane helixes. The amino acid residue aspartic acid (Asp)-113 participates in binding. The part of the receptor indicated in brown associates with G protein α-subunits. Two serine (Ser) residues in the intracellular carboxy-terminal tail are sites for phosphorylation by specific receptor kinases, which helps inactivate the receptor. (Adapted, with permission, from Frielle et al. 1989.)
The number of substances that act as second messengers in synaptic transmission is much fewer than the number of transmitters. Approximately 100 substances serve as transmitters; each can activate several types of receptors on the cell surface. The few second messengers that have been well characterized fall into two categories, intracellular and transcellular. Intra-cellular messengers are molecules whose actions are confined to the cell in which they are produced. Trans-cellular messengers are molecules that can readily cross the cell membrane and thus can leave the cell in which they are produced to act as intercellular signals, or first messengers, on neighboring cells.
A Family of G Proteins Activates Distinct Second-Messenger Pathways
The first G protein, Gs (where “s” stands for stimulatory), was identified more than 30 years ago by Martin Rodbell, Al Gilman, and their colleagues. Since that time a large family of G proteins has been identified. The G proteins are associated with the inner leaflet of the plasma membrane, where they interact with G protein-coupled receptors.
The G proteins that couple receptor activation to intracellular effectors are trimers that consist of three subunits: α, β, and γ (Figure 11–2). The α-subunit is only loosely associated with the membrane and is usually the agent that couples the receptor to its primary effector enzyme. The β- and γ-subunits form a strongly bound complex that is more tightly associated with the membrane. As we shall learn later in this chapter, the βγ complex of G proteins can also regulate the activity of certain ion channels directly.
Approximately 20 types of α-subunits have been identified, 5 types of α-subunits, and 12 types of γ-subunits. G proteins with different α-subunits couple different classes of receptors and effectors, and therefore have different physiological actions. The β-adrenergic receptor activates adenylyl cyclase by acting on Gs proteins; these contain the αs type of α-subunit. Some mus-carinic ACh receptors inhibit the cyclase by acting on Gi proteins (where “i” stands for inhibitory); these contain the αi type subunit. Still other G proteins (Gq/11 proteins, which contain αq – or α11-subunits) activate phospholipase C and probably other signal transduction mechanisms not yet identified. The Go protein, which contains the αo-subunit, is expressed at particularly high levels in the brain. Compared with other organs of the body, the brain contains an exceptionally large variety of G proteins. Even so, because of the limited number of classes of G proteins, one type of G protein can often be activated by different classes of receptors.
The known effector targets for G proteins are more limited than the types of G proteins. Important effectors include certain ion channels that are activated by the βγ complex, adenylyl cyclase in the cAMP pathway, phospholipase C in the diacylglycerol-inositol polyphosphate pathway, and phospholipase A2 in the arachidonic acid pathway. Each of these effectors (except for the ion channels) initiates changes in specific target proteins within the cell, either by generating second messengers that bind to the target protein or by activating a protein kinase that phosphorylates it. Despite their differences, second-messenger pathways activated by G protein signaling share a common design (Figure 11–5).
Figure 11-5 Synaptic second-messenger systems involving G protein coupling follow a common sequence. The signal transduction pathways illustrated here involve similar steps (left). Chemical transmitters arriving at receptor molecules in the plasma membrane activate a closely related family of G proteins (the transducers) that activate different enzymes or channels (the primary effectors). The activated enzymes produce a second messenger that activates a secondary effector or acts directly on a target (or regulatory) protein.
cAMP system. This pathway can be activated by a transmitter-bound β-adrenergic receptor, which acts through the Gs protein αs-subunit to activate adenylyl cyclase. Adenylyl cyclase produces the second messenger cAMP, which activates PKA. The G protein here is termed Gs because it stimulates the cyclase. Some receptors activate a G! protein that inhibits the cyclase.
Phosphoinositol system. This pathway, activated by a type 1 muscarinic acetylcholine (ACh) receptor, uses the Gq or G11 type of G protein (with α or α11-subunits, respectively) to activate a primary effector, phospholipase Cβ (PLCβ). This enzyme hydrolyzes the phospholipid, phosphatidylinositol 4,5-bisphosphate (PIP2), yielding a pair of second messengers: diacylglycerol (DAG) and inositol 1,4,5-trisphosphate (IP3). In turn, IP3 releases Ca2+ from internal stores, whereas DAG activates protein kinase C (PKC). The drop in membrane PIP2 levels can directly alter the activity of some ion channels.
Direct G protein-gating. This pathway represents the simplest synaptic mechanism for G protein-coupled receptor action. Acetylcholine (ACh) acting on type 2 muscarinic receptors activates the Gi protein, leading to functional dissociation of the α-subunit and βγ complex. The βγ complex interacts directly with a G protein-gated inward-rectifying K+ channel (GIRK), leading to channel opening and membrane hyperpolarization.
Hydrolysis of Phospholipids by Phospholipase C Produces Two Important Second Messengers, IP3 and Diacylglycerol
Many important second messengers are generated through the hydrolysis of phospholipids in the inner leaflet of the plasma membrane. This hydrolysis is catalyzed by three enzymes—phospholipase C, phospholipase D, and phospholipase A2—named for the ester bonds they hydrolyze in the phospholipid. The phospholipases each can be activated by different G proteins coupled to different receptors.
The most commonly hydrolyzed phospholipid is phosphatidylinositol 4,5-bisphosphate (PIP2), which typically contains the fatty acid stearate esterified to the glycerol backbone in the first position and the unsaturated fatty acid arachidonate in the second:
Activation of receptors coupled to Gq or G11 stimulates phospholipase C, which leads to the hydrolysis of PIP2 (specifically the phosphodiester bond that links the glycerol backbone to the polar head group) and production of two second messengers, diacylglycerol (DAG) and inositol 1,4,5-trisphosphate (IP3). DAG, which is hydrophobic, remains in the membrane when formed, where it recruits the cytoplasmic protein kinase C (PKC). PKC and DAG together with certain membrane phospholipids form an active complex that can phosphorylate many protein substrates in the cell, both membrane-associated and cytoplasmic (Figure 11–6A). Activation of some isoforms of PKC requires elevated levels of cytoplasmic Ca2+ in addition to DAG (Box 11-1).
Figure 11-6 Hydrolysis of phospholipids in the cell membrane activates three major second-messenger cascades.
A. The binding of transmitter to a receptor activates a G protein that activates phospholipase Cβ (PLCβ). This enzyme cleaves phosphatidylinositol 4,5-bisphosphate (PIP2) into the second messengers, inositol 1,4,5-trisphosphate (IP3) and diacylglycerol (DAG). IP3 is water-soluble and diffuses into the cytoplasm, where it binds to a receptor-channel on the smooth endoplasmic reticulum, the IP3 receptor, to release Ca2+ from internal stores. DAG remains in the membrane, where it activates protein kinase C (PKC). Membrane phospholipid is also a necessary cofactor for PKC activation. Some isoforms of PKC also require Ca2+ for activation. PKC is composed of a single protein molecule that has both a regulatory domain that binds DAG and a catalytic domain that phosphorylates proteins on serine or threonine residues.
B. The Ca2+/calmodulin-dependent protein kinase is activated when Ca2+ binds to calmodulin. The Ca2+/calmodulin complex then binds to a regulatory domain of the kinase, causing its activation. The kinase is composed of many similar subunits (only one of which is shown here), each having both regulatory and catalytic functions. The catalytic domain phosphorylates proteins on serine or threonine residues. (ATP, adenosine triphosphate; C, catalytic subunit; COOH, carboxy terminus; H2N, amino terminus; R, regulatory subunit.)
The second product of the phospholipase C pathway, IP3, stimulates the release of Ca2+ from intracellular membrane stores in the lumen of the smooth endoplasmic reticulum. The membrane of the reticu-lum contains a large integral membrane macromol-ecule, the IP3 receptor, which forms both a receptor for IP3 on its cytoplasmic surface and a Ca2+-permeant channel that spans the membrane of the reticulum. When this macromolecule binds IP3 the channel opens, releasing Ca2+ into the cytoplasm (Figure 11-6A).
The increase in intracellular Ca2+ triggers many biochemical reactions and opens calcium-gated channels in the plasma membrane. Calcium can also act as a second messenger to trigger the release of additional Ca2+ from internal stores by binding to another integral protein in the membrane of the smooth endoplasmic reticu-lum, the ryanodine receptor (so called because it binds the plant alkaloid ryanodine, which inhibits the receptor; in contrast, caffeine opens the ryanodine receptor). Like the IP3 receptor to which it is distantly related, the ryanodine receptor forms a Ca2+-permeant channel that spans the reticulum membrane; however, cytoplasmic Ca2+, not IP3, gates the ryanodine receptor-channel.
Calcium often acts by binding to the small cytoplas-mic protein calmodulin. An important function of the calcium/calmodulin complex is to activate the Ca2+/calmodulin-dependent protein kinase (CaM kinase). This enzyme is a complex of many similar subunits, each containing both regulatory and catalytic domains within the same polypeptide chain. When the Ca2+/calmodulin complex is absent, the C-terminal regulatory domain of the kinase binds and inactivates the catalytic portion. Binding to the Ca2+/calmodulin complex causes conformational changes of the kinase molecule that unfetter the catalytic domain for action (Figure 11-6B). Once activated, CaM kinase can phosphorylate itself through intramolecular reactions at many sites in the molecule. Autophosphorylation has an important functional effect: It converts the enzyme into a form that is independent of Ca2+/calmodulin and therefore persistently active even in the absence of Ca2+.
Persistent activation of protein kinases is a general and important mechanism for maintaining biochemical processes underlying long-term changes in synaptic function associated with certain forms of memory. In addition to the persistent activation of Ca2+/calmodu-lin-dependent protein kinase, PKA can also become persistently active following a transient increase in cAMP, because of the enzymatic degradation of its regulatory subunits through the ubiquitin pathway. The decline in regulatory subunit concentration results in the long-lasting presence of free catalytic subunits, even after cAMP levels have declined, leading to the continued phosphorylation of substrate proteins. PKC can also become persistently active through proteolytic cleavage of its regulatory and catalytic domains or expression of a PKC isoform that lacks a regulatory domain.
Hydrolysis of Phospholipids by Phospholipase A2 Liberates Arachidonic Acid to Produce Other Second Messengers
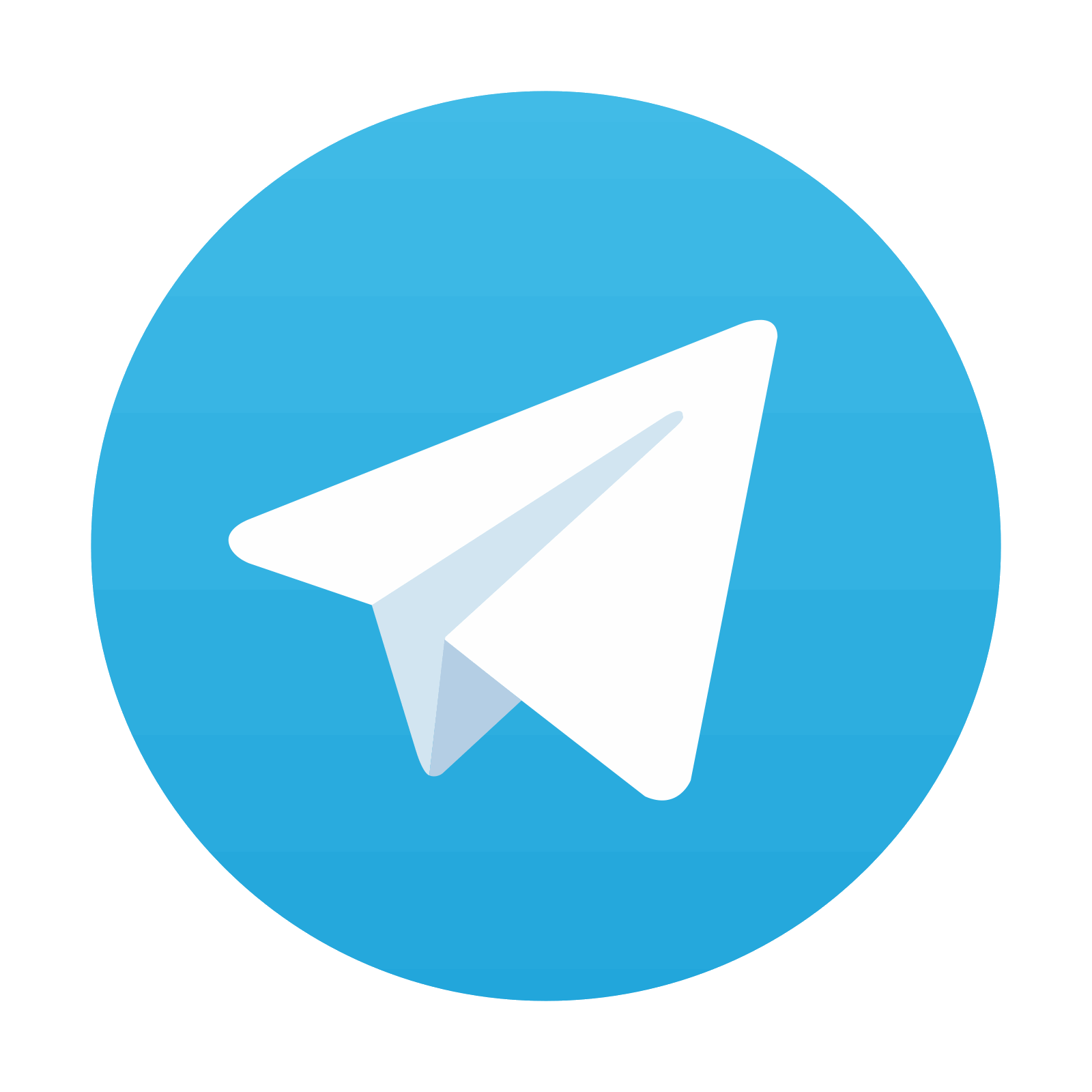
Stay updated, free articles. Join our Telegram channel
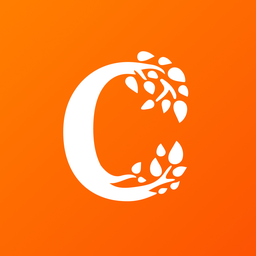
Full access? Get Clinical Tree
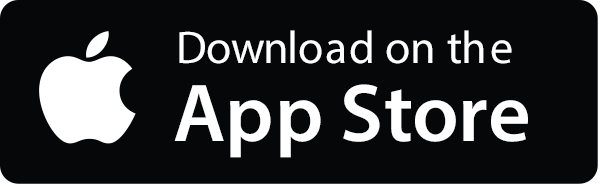
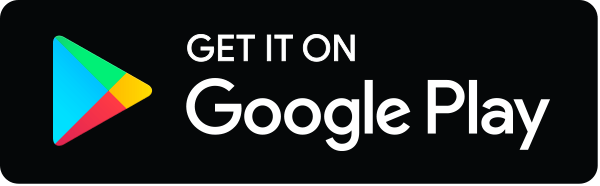