Introduction
Knowing the extent of brain damage in the context of acute cerebral injury can be of paramount importance, especially when the patient is at crossroads, when a decision should be made about whether or not to continue care. Although clinical or electrophysiologic examinations provide some information, they are not always reliable because of the degree of sedation these patients receive. Computed tomography (CT) is the first line of imaging examination to rule out a neurosurgical emergency (e.g., extra- or subdural hematoma) or suggest increased intracranial pressure (ICP). However, CT cannot provide a reliable evaluation of the prognosis because of its poor sensitivity (especially in the posterior fossa) and lack of specificity. Magnetic resonance imaging (MRI) has excellent sensitivity to demonstrate brain lesions and characterize different pathologic processes and so can be the neuroimaging procedure of choice in many patients. This chapter reviews MRI brain imaging, MRI findings in select pathologies encountered in the neurocritical care unit (NCCU) (e.g., traumatic brain injury, stroke, infection, anoxic injury, and hemorrhage among others), and use of MRI including advanced techniques in estimating prognosis. MRI imaging of the spine, extracranial vasculature, or other organ systems (e.g., cardiac MRI) is beyond the scope of this chapter, although all can be relevant to patient care.
MRI in a Brain-Injured Patient: the Procedure
Contraindications and Safety Rules
Performing an MRI in a critically brain-injured patient is a challenging task. The MRI scanner is mostly a very powerful magnet (1.5-3 tesla), inside which the subject is almost completely inaccessible during the 30- to 60-minute examination. There are several consequences of these technical hurdles:
- ▪
All medical devices associated with the patient must be MR compatible. In general, since 1995 implantable devices (e.g., stents, cardiac valves, neurosurgical clips, or coils among others) are almost always MR compatible at 1.5 tesla. At higher field strength, however, their safety has not been established. MR examinations often are possible at 1.5 tesla in patients with pacemakers, neurostimulators, and implantable defibrillators but remain dangerous, and require extra caution. The presence of ferromagnetic metals inside the eye or central nervous system, such as older aneurysm clips, is a contraindication to MRI.
- ▪
Patient monitoring is hampered because only limited visual observation of the subject is possible, and the monitoring devices, designed for use in a high magnetic field are not as reliable as their counterparts used in the intensive care unit (ICU). Thus MRI examination should not be attempted in respiratory or hemodynamically unstable patients, unless the procedure is absolutely needed.
- ▪
The MRI examination should not be attempted in patients with intracranial hypertension because a prolonged flat position can increase ICP.
- ▪
Electric syringes do not operate properly in the magnetic field and have to be located away from the patient and the magnet. This requires long intravenous (IV) tubing that may slow or alter drug or fluid delivery.
- ▪
MRI contrast media injections should be avoided in patients with severe renal insufficiency because this may lead to nephrogenic systemic fibrosis.
MRI Sequences
An ever-increasing number of sequences can be performed to examine the brain. Because the duration of the examination is limited, great care should be taken to select the most appropriate sequence for the clinical question. Routinely performed MRI sequences include the following:
- ▪
T1-weighted sequences are the “anatomic” sequences. They are used mostly to detect morphologic changes, such as ventricle enlargement or brain atrophy, and to detect subacute parenchymal blood (methemoglobin, areas of high signal) and areas of contrast enhancement.
- ▪
T2-weighted sequences are used to demonstrate lesions (contusions, ischemia, edema), which generally appear as areas of increased signal. In neuroimaging the most widely used T2-weighted sequence is the fluid-attenuated inversion recovery (FLAIR) sequence in which the signal of the cerebrospinal fluid (CSF) is suppressed. In addition to its superb ability to demonstrate areas of parenchymal increased signal, FLAIR plays an important role in demonstrating acute subarachnoid hemorrhage (SAH).
- ▪
T2*-weighted sequences are specially designed to demonstrate intraparenchymal blood, both acute (deoxyhemoglobin) and chronic (hemosiderin), which appears as areas of decreased signal, the latter persisting for years.
- ▪
MR angiography (MRA) can be performed to examine the arteries of the circle of Willis or MR venography (MRV) to evaluate the intracranial veins and sinuses.
- ▪
Diffusion-weighted imaging studies the random movements of water molecules. It is represented as the apparent diffusion coefficient (ADC), which is a physical characteristic of the tissue. Only a very limited number of pathologic processes can decrease the ADC, the most widely encountered being acute ischemia.
These sequences are available on all MR scanners and can be routinely performed. More advanced acquisitions, where available, can be used to better characterize the pathologic processes and the extent of brain damage. Indeed, normally appearing brain tissue on T2-weighted sequences can be either normal or abnormal according to what other sequences are used:
- ▪
Diffusion tensor imaging (DTI) is a variant of diffusion imaging that studies the spatial restriction of diffusion. The presence of white matter tracts “channels” the water molecules, which move in a preferential direction. The preferential restriction of diffusivity is measured by the fractional anisotropy (FA). This sequence can also be used to follow individual white matter tracts, such as the pyramidal and spinothalamic. This processing is called fiber tracking .
- ▪
MR spectroscopy (MRS) studies brain metabolism. This sequence quantifies molecules specific to some cell types or physiologic processes that may assist in differentiating brain tumors from radiation necrosis or from an abscess among others. The most easily detected are as follows:
- ▪
N-acetyl aspartate (NAA) is a neuronal marker synthesized in the mitochondria of neurons from the amino acid aspartic acid, which decreases where there is neuron damage or death. For example, a reduction in NAA is observed after stroke reaching a nadir about 2 weeks after symptom onset.
- ▪
Creatine (Cr), a ubiquitous molecule involved in energetic metabolism. It is often used as a reference.
- ▪
Choline (Cho) increases in cases of membrane synthesis (e.g., brain tumors) or catabolism.
- ▪
- ▪
Blood oxygen level–dependent (BOLD) functional MRI (fMRI) is less commonly used because it is difficult to perform in a sedated patient. It has mostly been used after the acute phase, in vegetative or minimally conscious patients, to search for the presence of residual high order functional activities. The coherent spontaneous BOLD fluctuations, observed with the resting state fMRI method, which do not require the patient’s cooperation, have been shown to be still present under general anesthesia and could possibly be useful to evaluate the persistence of structured brain activities in comatose patients.
MRI of Major Pathologies
Ischemic Stroke
Acute ischemic stroke (AIS) rarely alters consciousness, except in massive hemispheric infarcts with brain herniation or basilar artery occlusion. However, the diagnosis of AIS should be considered when an ICU patient develops a new neurologic deficit, especially if an early CT scan is normal or precipitating factors such as cardiac or vascular surgery are present, or a brainstem or posterior fossa stroke is likely. MRI is the examination of choice to confirm the diagnosis. A rapid (less than an hour after symptom onset) decrease of the ADC of the affected brain area is seen on MRI. FLAIR is normal within the first 4 to 6 hours and then shows areas of increased signal. MRA may demonstrate the level of vascular obstruction. The presence of an area of decreased ADC and normal FLAIR is highly sensitive and specific of AIS ( Fig. 28.1 ). MRI findings in acute stroke are listed in Table 28.1 .

|
The gold standard imaging technique to assess cerebral hemodynamics (i.e., flow and metabolism) is positron emission tomography (PET) (see Chapter 29 ), but novel MRI approaches provide methods to measure cerebral blood flow (CBF), cerebral blood volume (CBV), and cerebral metabolic rate of oxygen (CMRO 2 ).
- ▪
When perfusion-weighted imaging (PWI) is used with diffusion-weighted imaging (DWI), the ischemic penumbra often can be identified. In PWI, protons in arterial blood to the brain are magnetically tagged; this creates an intra-arterial contrast with the intracranial contents and so allows for calculation of perfusion maps (i.e., cerebral blood flow). PWI is relatively time consuming to obtain, but a PWI-DWI mismatch in which there is still viable tissue but inadequate flow identifies tissue at risk for infarction. This information can be used to guide thrombolytic therapy. Alternatively, a DWI-FLAIR mismatch can be used to guide thrombolytic therapy. Compared with PET studies MRI evaluation may overestimate the size of the penumbra.
- ▪
Advances in phase contrast MRA allow noninvasive assessment of blood flow rates and flow direction through quantitative magnetic resonance angiography (qMRA). This permits evaluation of CBF and collateral circulation in patients with stroke, transient ischemic attacks (TIAs), or occlusive cerebral vascular disease, or after bypass surgery.
- ▪
Arterial spin labeling (ASL) MRI, including pulsed ASL (PASL) or continuous ASL (CASL), remains the most popular MRI method used to measure CBF. ASL does not require exogenous contrast and is best used to measure CBF in the cerebral cortex. Results are less robust in white matter. A newer ASL technique, vessel-selective ASL (VS-ASL) permits separate labeling of feeding arteries such that perfusion territories can be measured.
- ▪
CBV can be measured by modified ASL approaches. In addition, CBV may be noninvasively measured using vascular space occupancy (VASO) MRI and its variant “inflow VASO” (iVASO), or through quantitative STAR labeling of arterial regions (QUASAR).
- ▪
Decisions about CBF require an understanding about metabolism (CMRO 2 ). For example, brain tissue remains viable when CMRO 2 is preserved through increased oxygen extraction fraction (OEF) even when CBF is reduced. OEF is best evaluated using PET, but BOLD provides an indirect marker of neuronal activity and so can provide qualitative results that relate CBF and CMRO 2 in patients with chronic cerebrovascular disease. More research is required before this technique can be applied in a quantitative fashion to patients in the ICU.
Intracranial Hematoma
CT scan is the imaging modality of choice to diagnose an acute intracranial hematoma. However, MRI can be performed as a diagnostic tool to determine the etiology of the intracranial hematoma and evaluate the extent of surrounding edema or as a prognostic tool to determine the extent of brain injury (see later section on The Prognostic Value of MRI ). Hemorrhagic lesions are best detected with T2*-weighted sequences, on which they appear as areas of decreased signal. This sequence also may show associated small hypointensities scattered in the brain (or “blebs”), resulting from previous microvascular injuries. FLAIR sequences can help define the extent of other vascular risk factor–related brain lesions (e.g., lacunar infarcts and leukoaraiosis).
Anoxic Brain Injury
Anoxic brain injury, for example cardiac arrest, is common. Lesions can involve the pallidum, the cerebellum, the thalamus, the striatum, and the cortex (laminar necrosis), more specifically the occipital and perirolandic areas ( Figs. 28.2 and 28.3 ). The brainstem usually is spared. Lesions appear as hyperintense first on FLAIR and diffusion imaging (usually a decreased ADC), then on T1 sequences. The time course of these changes is still poorly known; they can appear within the first 24 hours or be delayed for as much as 1 week. The presence of hyperintensity in the cortex (particularly the motor cortex) and striatum suggests poor neurologic prognosis. Diffusion MRI also may help predict long-term outcome after cardiac arrest. This can be particularly valuable when induced hypothermia is used. In the most severe forms, brain atrophy is observed in the following weeks. MR spectroscopy, if performed early, may show the presence of lactate. Later, a decrease in NAA can be observed (see Fig. 28.3 ). Its prognostic value has been well established in children, especially in cases of near drowning and perinatal anoxia. Susceptibility-weighted imaging also may be used to help predict outcome after neonatal hypoxic-ischemic injuries.


Subarachnoid Hemorrhage
CT is considered the imaging modality of choice to detect acute SAH. MRI may be useful, depending on what sequences are performed to diagnose SAH if CT is negative and in the subacute phase. The presence of blood in the subarachnoid spaces gives a hyperintensity on the FLAIR sequence. False negatives may occur and a normal examination does not rule out the diagnosis, especially if the MRI is performed several days after the onset of symptoms because of CSF washing of blood. In addition the diagnostic accuracy of FLAIR may be reduced when the SAH is in compressed cisterns. Higher-field magnets (3 tesla and more) tend to have a higher rate of both false positives and negatives. MRA or MRI can be used to demonstrate the causative aneurysm and can help evaluate ischemic complications secondary to vasospasm. The results of MRA are most accurate when there is severe arterial narrowing but less reliable for moderate or mild vasospasm. In addition, the dynamics of blood flow are altered when there are multiple areas of vessel narrowing; this compromises the accuracy of time-of-flight sequences. Finally, admission DWI imaging may help guide management decisions in poor-grade SAH patients; those with no or only spotty DWI lesions tend to have a good outcome.
Infectious Brain Diseases
MRI can be extremely useful in some infectious brain diseases.
- ▪
The lesions of herpetic meningoencephalitis predominate in the temporal lobes, insula, and basi-frontal areas. Contrast-enhanced, ischemic or hemorrhagic foci may be present.
- ▪
Bacterial meningitis usually does not require an MRI examination. This examination is sometimes performed in patients who develop focal signs or altered consciousness, in search for an abscess, ventriculitis, or vasculitis.
- ▪
Brain abscesses appear as cystic, contrast-enhancing lesions. The capsule shows hypointensity on T2 or FLAIR and slight hypersignal on T1-weighted sequences, the content of the abscess has decreased ADC, and amino acids can be detected on MR spectroscopy ( Fig. 28.4 ).
Fig. 28.4
This 45-year-old patient with a history of intravenous drug abuse and alcoholic cirrhosis presented with fever and a rapidly altering consciousness.
Magnetic resonance imaging (MRI) images: T1 with gadolinium (A), fluid-attenuated inversion recovery (FLAIR) with gadolinium (B), diffusion-weighted sequences (C), and apparent diffusion coefficient (ADC) map (D). MR spectroscopy at short (E) and long (F) echo times. The contrast-enhancing cystic lesion is high signal on diffusion imaging with a decreased ADC, and the presence of amino acids (Aa) on MR spectroscopy are typical of an intracranial abscess. The associated meningitis and ventriculitis are diagnosed by the presence of increased signal of the sulci and the frontal horns of the lateral ventricles on the FLAIR sequence.
- ▪
Both edematous and ischemic lesions of the white matter may be seen in septic shock–induced brain dysfunction. However, the potential prognostic value of MRI in this context remains to be fully elucidated.
Posterior Reversible Leukoencephalopathy Syndrome
Posterior reversible leukoencephalopathy syndrome (PRESS) is a complication of uncontrolled hypertension and of some treatments such as immunosuppressors or some chemotherapeutic agents (e.g., intrathecal methotrexate). Its diagnosis is easily made with MRI, which shows areas of subcortical edema that predominate in the posterior areas. The lesions also can involve the frontal white matter or posterior fossa. Contrast enhancement is uncommon. Diffusion imaging should be performed to evaluate PRESS: two different patterns can be observed :
- ▪
Areas of increased ADC correspond to vasogenic edema. These lesions usually are reversible and associated with good prognosis.
- ▪
Areas of decreased ADC correspond to cytotoxic edema; these lesions generally are not reversible and suggest a more reserved prognosis.
Traumatic Brain Injury
CT is the initial imaging study for traumatic brain injury (TBI) because it permits rapid and accurate diagnosis of intracranial hematoma and mass effect on which to base management decisions. MRI is useful for follow-up evaluation especially of diffuse axonal injury (DAI), examination of brainstem pathology, and to aid in decisions about prognosis. Newer MRI sequences (e.g., gradient-recalled echo, susceptibility-weighted imaging (SWI), and DWI) have greater sensitivity for diffuse brain injury, whereas diffusion tensor imaging provides information about white matter injury. Multiple mechanisms lead to cerebral injury in TBI; hence the imaging pattern can be varied and heterogeneous. This chapter focuses on pathology observed in the brain and what is most relevant to follow up during ICU care. The reader is referred to recent reviews for a full discussion of imaging in TBI including of the skull and craniofacial structures and for the common data elements on TBI imaging.
- ▪
Cerebral contusions can be edematous or hemorrhagic. They appear as areas of hyperintensity on the FLAIR sequence, with associated hypointensity on the T2* sequence when hematomas are present. Their most frequent locations are the basi-frontal areas and the temporal lobes.
- ▪
DAI ( Fig. 28.5 ). This injury results usually from deceleration and is associated with shearing of the white matter fibers. Three different kinds of DAI can be observed:
- ▪
Hemorrhagic DAI is the most easily detected: the white matter lesions are associated with lesions of the small arteries, causing very small areas of bleeding, which give hypointense dots (or blebs) on the T2* sequence. They have been associated with persistent cognitive impairment in patients with an otherwise normal MRI. SWI is a very sensitive method to identify microbleeds, which are a marker of traumatic axonal injury.
- ▪
Ischemic DAI occurs when the lesions of the small arteries lead to vascular occlusion and ischemia. They are best detected with the diffusion sequence that shows punctiform areas of decreased ADC.
- ▪
DAI without vascular lesions is the most difficult to detect. These lesions do not give any sign on MR sequences, except atrophy of relatively late onset. DTI is probably the best method to detect them; interruption of the white matter tract leads to a decrease in the fractional anisotropy of the area, or can be detected by fiber tracking. However, although some studies have shown marked difference in fractional anisotropy values between TBI subjects with a good and poor prognosis, the role of DTI to determine prognosis of individual patients remains to be fully defined.
- ▪
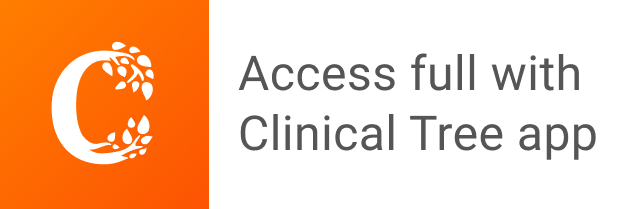