Fig. 6.1
Invasive multimodality monitoring “bundle” in a patient in the neurointensive care unit. (a) External view of the patient’s head with microdialysis and rCBF probes in the foreground and the Licox probe (PbtO2, Temperature, ICP) in the background. (b) X-ray skull survey with right frontal insertion of probes shown in (a). (c) CT head without contrast showing the tips of the probes (red arrow) immediately next to each other. (d) Conventional Angiography showing MMM probes in relation to the cerebral vasculature in the right frontal ACA/MCA territory. rCBF regional cerebral blood flow, PbtO2 partial brain oxygen pressure, ICP intracranial pressure , MMM multimodality monitoring, ACA anterior cerebral artery, MCA middle cerebral artery
The physiologic PbtO2 range is still a matter of debate. Based on data obtained in patients undergoing elective neurosurgical procedures and in patients with acute brain injuries, 20–35 mmHg can be considered normal, <20 mmHg moderate hypoxia, and <10 mmHg severe hypoxia [42–44]. There is an abundance of data showing that very low PbtO2 values correlate with poor outcome, in both TBI and SAH patients [45–48]. Further evidence for the physiologic plausibility of PbtO2 measurement was provided by a study correlating PbtO2 and regional cerebral blood flow [49] and by a study showing that PbtO2 in brain dead patients is zero mmHg [50]. So far, PbtO2-targeted therapy and its impact on outcome have only been studied in small patient cohorts but with some promising results [51].
Cerebral Microdialysis
MD allows the determination of interstitial molecule concentrations in the brain, by insertion of a catheter–with attached tubing system for filtering and passage of the dialysate–into the white matter [52, 53]. The dialysate is then typically analyzed at the bedside [54]. The maximal sampling frequency is limited to approximately 6 times per hour due to the time needed for equilibration of the chemical molecule gradient across the microdialysis membrane. Most institutions that use MD on a routine basis sample the catheter fluid once per hour, which may be a limitation when MD parameters are to be compared to other monitoring modalities with higher time resolution.
Molecules of interest include brain glucose, the glycolysis products lactate and pyruvate, as well as markers of excitotoxicity or cell damage, such as glutamate and glycerol [55]. The lactate-pyruvate ratio (LPR) rather than the two parameters in isolation is believed to be associated with tissue energy failure . A low interstitial brain glucose concentration in combination with a high LPR can be indicative of hypoxia/ischemia. However, elevation of the LPR alone may indicate an accelerated brain metabolism and is not necessarily associated with brain damage. MD-values need to be interpreted in context; the comparison with PbtO2 and rCBF has been shown to provide a meaningful first approach to evaluate the metabolic state of the brain tissue [56, 57].
Normal glucose values range between 1.5 and 2 mM. LPR values of greater than 25 and 40, respectively, have been described as a marker of injury [58–60]. Normal ranges of glycerol and glutamate—markers of brain cell membrane break down and excitotoxicity—are less well established. Generally glutamate concentrations <10 mM and glycerol concentrations <50 mM are considered normal [54, 61–63].
Both low glucose and high LPR are associated with poor outcome [17, 56, 57]. Moreover high interstitial glutamate in SAH patients has been shown to indicate delayed cerebral ischemia [64]. In a large observational study including 223 patients with TBI, glucose and LPR contralateral to traumatic injury were each independently associated with 6-month mortality [59].
Although brain glucose metabolism can be altered by systemic interventions [65], to date, no interventional studies using MD-values as treatment targets to improve clinical outcome have been conducted. However, MD with PbtO2 measurements have been used to identify optimal MAP/ICP and hemoglobin values, which may indirectly impact clinical outcome [26, 66, 67].
EEG as a Component of Multimodality Monitoring
Surface EEG
In the neuro ICU several methods of EEG recording can be applied to obtain EEG data from patients with acute brain injury, among them spot EEG, repeated short EEG recordings, continuous surface EEG, and depth EEG (dEEG). All of these modes of EEG recording can be enhanced by using quantitative EEG (see Chap. 20 for details). Surface EEG (sEEG), as one of the oldest and most intensely studied techniques of monitoring the brain, has its unquestioned diagnostic value in seizure detection, monitoring the depth of sedation, and in prognostication [68]. Open questions in the neuro ICU context include the choice of EEG technique (or combination of techniques) and duration of EEG recording that produces the highest diagnostic yield in a given disease entity and patient [69]. In recent years, technical advancements have made digital transformation of the analog EEG signal, storage, and—at least partly—the automated analysis of large amounts of EEG data possible. Nevertheless, continuous EEG (cEEG) recordings still require a substantial amount of manpower at significant cost, which is why research on effective use is warranted.
Both through modifications of the EEG recording technique and through development of data analysis methods researchers have tried to improve the applicability of sEEG in the neuro ICU [70].
Quantitative EEG (for an Extensive Discussion on qEEG Please Refer to Another Chapter of the Book)
A relatively new development in the neuro ICU is the use of quantitative EEG (qEEG) to aid in the timely extraction of diagnostic information from the large amounts of cEEG data. Various algorithms are available to analyze and visualize qEEG data. After undergoing Fast Fourier Transformation the raw EEG data can either be represented as total power, ratios of power (e.g., alpha delta ratio), or spectrograms. Spectrograms in turn can be graphed as numbers, compressed spectral arrays (CSA), staggered arrays, or histograms [71, 72]. The analogous interpretation of raw EEG data is too time consuming to be feasible by a neurointensive care physician alone whose responsibilities will only grow with the technical progress in the field of neurocritical care , leaving less time for EEG review. The alternative, employment of full time electrophysiologists, is a financial and logistic challenge that most hospitals are not able to afford. In the future, qEEG will hopefully offer efficient screening and alarm mechanisms indicating clinically concerning EEG trends, in order to make big EEG data accessible and useful at the bedside [73].
Automated Seizure Detection Algorithms
Another approach to master the task of extracting relevant information from large amounts of raw EEG recordings is the development of seizure detection software. For such software to detect seizures with reasonable sensitivity and specificity, seizures should ideally have a clear on- and offset and should be clearly distinguishable from the EEG background. While this is often the case in a relatively noise-free environment, such as epilepsy monitoring units, it is rarely the case in neurointensive care units that have many ambient sources of noise [74, 75]. Moreover, muscle artifacts and periodic discharges are more common in the NICU. The latter share many electrographic features with seizures and are therefore difficult to distinguish by automated seizure detection software [76, 77]. Indeed there is ongoing debate regarding the clinical relevance of periodic discharges that fall along the ictal-interictal spectrum lacking the discrete on–offset of events that are universally recognized as ictal. Seizure detection algorithms using intracortical EEG data have not been tested so far, but are promising given the overall high signal to noise ratio of dEEG.
Depth EEG
Surface EEG has the advantage of providing a global electrophysiological signal covering most parts of the cerebral cortex. However, sEEG electrodes are separated from the source of the EEG signal originating from the brain tissue by several structures: skin, hair, the skull, as well as the meninges and any pathologic material (blood, pus, etc.) that may lie within. Distant from the source of the signal, sEEG is also close to sources of noise. Sources of noise include poor electrode contact and—particularly in the neuro ICU–surrounding electronic devices, which may cause artifacts distorting the EEG signal. Another major source of artifact is the electrical signal generated by muscle and movement. These disadvantages may be overcome by the local insertion of intracortical electrodes, which allows at least local—not global—EEG recordings with minimal artifact and a high signal to noise ratio.
Invasive EEG monitoring as a diagnostic modality arose in the context of seizure focus localization in patients with treatment-refractory focal epilepsy. It is typically achieved either by subdural/epidural grid placement or by implantation of intracortical strip or depth electrodes [78], which share a similar risk profile with other invasive monitoring techniques [79]. Despite comparatively poor spatial resolution, intracortical electrodes may prove to be a valuable diagnostic addition to sEEG monitoring in neurointensive care units that routinely use depth electrodes [79, 80].
EEG and Multimodality Monitoring
The concurrent use of EEG with other MMM techniques provides the opportunity to get a more complete picture of ongoing brain physiology, during major pathologic events like seizures, delayed cerebral ischemic events after SAH, or during waves of cortical spreading depression [81–83].
Apart from a few recent studies data are lacking using a combined EEG/MMM methodology [80, 84–86]. One of the most promising areas of research is the characterization of ongoing physiology during seizures, which may potentially help find treatment targets beyond administration of anticonvulsant drugs, with the goal to improve physiology. Seizures in ICU populations are both very common and associated with worse outcomes [85, 87], which is why the characterization of the physiologic signature of seizures through the use of MMM is very promising. With the use of cEEG electrographic seizures have been detected in up to 15% of patients with spontaneous SAH [87, 88], in 15% of patients with post-cardiac arrest syndrome [89], in around one quarter of patients with intracerebral hemorrhage [90, 91], in up to one third of patients with TBI [92, 93] and in one third of patients with CNS infections [94]. By the same token, 16 and 10% of patients in surgical and medical ICU populations are found to have electrographic only seizures [95, 96]. Better understanding of the underlying pathophysiological processes occurring during acute brain injury seizures may allow identification of seizures that deserve more or less aggressive treatment. Lastly, through the combined use of EEG and MMM the so-called ictal-interictal continuum might be characterized further, distinguishing EEG patterns that warrant treatment from those that do not [97].
Multimodality Monitoring Signature of Seizures
The detection of electrographic seizures is important because studies suggest a negative influence of seizures on clinical outcome [85, 87, 98]. A study in 479 SAH patients, undergoing cEEG monitoring using sEEG electrodes in the vast majority of cases, suggested a vicious cycle between seizures, inflammation, and poor outcome following acute brain injury [98]. However, the changes of physiology and metabolism of brain tissue during seizures remain poorly characterized, which is why treatment of seizures is restricted to administration of anticonvulsant drugs. MMM during seizures has the potential to generate a more complete picture of brain tissue changes and eventually open up new therapeutic options that target restoring physiology rather than seizure cessation.
Animal Studies
In animal models researchers have investigated the MMM signature of seizures for decades using varying techniques to measure ICP, CPP, cerebral blood flow , brain oxygen , and cerebral metabolites before, during, and after seizures [99]. Animal models have the advantage of being “clean” models of seizure physiology in contrast to human patients in the neurointensive care unit who tend to have a severe underlying brain pathology and systemic effects such as pneumonia or sepsis to account for [100]. Despite variability among studies regarding the type of animal model, the mode of seizure induction, and the methods employed (including variable sites of MD catheter placement) some comparable results can be extracted, indicating a potential common MMM signature of seizures.
As early as 1939, Penfield and co-workers described an increase of cerebral blood flow during seizures [101] and this finding has been reproduced quite consistently across animal species and seizure models using different types of intraparenchymal probes to measure rCBF [102–105].
Conversely brain tissue oxygen tension eventually decreases with ongoing seizure activity, sometimes following an initial increase of local oxygen tension, indicating a possible initial compensatory effort which eventually ends with decompensation of oxygen delivery [106–109].
Although not consistently reproduced across different disease processes, there are some animal studies showing that prolonged seizures may cause ICP elevations [110, 111]. Consequently CPP may be reduced during seizure activity, unless increased blood pressure during the event compensates the ICP elevation. However CPP is also influenced by various other factors such as presence or absence of functioning autoregulation; therefore, CPP changes during seizures are difficult to investigate and have not been systematically studied in animal models [84].
During experimentally induced status epilepticus initially marked increases in extracellular glucose and lactate levels have been observed, indicating increased energy demand and turnover [112]. This hypermetabolic situation may eventually lead to energy failure as indicated by decreases of extracellular glucose and increases in the LPR after 20–40 minutes of ongoing status epilepticus, as observed in the L-homocysteine induced seizure model in rats [113]. Marked increases of extracellular glutamate and glycerol after prolonged seizure activity have been interpreted as indicative of cell death as a consequence of prolonged status epilepticus [112, 114–118].
Studies in Humans
MMM studies in humans often face criticism because data is heterogeneous and not always conclusive, perhaps not surprising as most studies include heterogeneous disease processes and disease severities. In clinical practice all efforts are being made to terminate seizures as early as possible. However, a clear picture of MMM changes during seizure activity can often only be seen after prolonged status epilepticus, as evident from the animal studies described above. Figure 6.2 illustrates typical changes of brain oxygen and perfusion preceding, during, and after a prolonged electrographic seizure in a patient with a high-grade spontaneous subarachnoid hemorrhage (Fig. 6.2).


Fig. 6.2
Multimodality monitoring signature of an electrographic seizure. (a) rCBF, PbtO2, CPP, and HR relative to the onset of a seizure, detected on both surface and depth EEG. The background colors in (a) indicate (1) white = no seizure activity, (2) lgiht grey = ictal-interictal continuum, (3) dark grey = seizure. B1–B3, Raw EEG clips (16 s each) corresponding to the phases shown in (a). Panel B1 shows no seizure activity, B2 ictal-interictal continuum, B3 seizure. rCBF regional cerebral blood flow, PbtO2 partial brain oxygen tension, CPP cerebral perfusion pressure, HR heart rate
Apart from the dynamics of MMM parameters, secondarily developing lesions are often encountered following acute brain injury such as ischemic and hemorrhagic lesions and will affect the recorded brain physiology. Pathophysiologic changes of primary injury may at times be difficult to differentiate from secondary events. Moreover, variable disease severity at baseline is likely reflected in different baseline MMM values which is hypothetically the reason why pooled data is less likely to produce statistically significant results than comparison of within-subject changes [81, 85]. Despite these “real life” limitations a decent body of scientific evidence has been generated describing MMM changes during seizure activity in human patients.
Ictal hyperperfusion is a well-studied phenomenon, and has been seen in numerous studies using positron emission tomography, CT perfusion, MR perfusion, and Xenon CT in patients with and without acute brain injury, but studies using invasive rCBF measurement are sparse [119, 120]. In line with animal data, one study found that rCBF during seizures increases with continuous seizure activity, after approximately 10 minutes [85]. Also consistent with the animal data, PbtO2 has been found to decrease during seizures prior to the increase of rCBF, suggesting that perfusion may increase as a compensatory reaction to hypoxia [85]. In a group of 10 TBI patients with seizures compared to a matched control group of TBI patients without seizures, the occurrence of seizures correlated with a prolonged sustained increase of ICP [121]. In the study by Claassen et al. electrographic seizures were accompanied by an immediate increase of ICP, CPP, heart rate, MAP, respiratory rate, and minute ventilation [85]. These changes in cardiovascular parameters are common in partial and generalized epilepsies and are consistent with previous studies [122, 123].
Few studies are available evaluating brain MD changes during seizure activity in humans. In 30 subjects with TBI the occurrence of electrographic seizures coincided with a decrease of extracellular glucose concentrations [124] and this finding was reproduced in a recent study in 29 TBI patients in whom extracellular glucose concentrations during seizures were significantly lower than at baseline (mean 0.8 vs. 1.7 mmol/l) [81]. Administration of systemic insulin lowers both systemic and brain extracellular glucose concentrations and correlates with markers of metabolic stress in the brain, namely increased LPR and increased glutamate concentrations [65, 125]. The few available studies investigating lactate and LPR during seizures are in line with these findings . A study by During and colleagues investigated the effect of spontaneously occurring seizures in (otherwise healthy) patients with treatment-refractory complex partial seizures, after bilateral implantation of MD catheters in both hippocampi. Partial seizures with secondary generalization were accompanied by a mean 90% lactate increase in the first 60–90 minutes after seizure onset. This increase was restricted to the side of seizure initiation [126]. Pyruvate concentrations were not determined in this study.
MD changes may last beyond seizure cessation. In a study of non-convulsive seizures in TBI, Vespa et al. found prolonged and significantly higher LPR values in the seizure group compared to the group without seizures. This effect lasted beyond 100 hours after admission [121]. However, this finding was not reproduced in the study by Claassen et al., who did not find significant changes of MD parameters related to the occurrence of electrographic seizures. The discrepancy between both papers is possibly due to the statistical methods employed or due to the different entities studied (TBI versus SAH) [127]. In a recent study in patients with severe TBI undergoing MMM including MD, the comparison of 12 patients with seizures and 8 patients without seizures showed a numerically higher hourly LPR burden in the seizure group (as quantified using the area under the curve). This finding was not statistically significant in a pooled analysis but reached significance using a within-subject-design analyzing LPR changes of single patients on an hour by hour basis [81].
Extracellular glutamate is a key factor in abnormal neuronal discharges and has been implicated in triggering and maintaining seizures, and in kindling epilepsy [128]. MD studies in patients with temporal lobe epilepsy have shown chronically increased extracellular glutamate concentrations on the side of the epileptic focus leading to excitotoxicity, which hypothetically entertains the increased likelihood of further seizures [129]. Ronne-Engström and colleagues investigated extracellular amino acid levels during seizures and during the period immediately following the seizures in seven patients with epilepsy of focal origin by implanting a MD device into the epileptic focus. Extracellular glutamate concentrations rose approximately sevenfold during spontaneously occurring seizures, but normalized immediately after [130]. In patients with TBI, seizures were associated with the occurrence of transient extracellular glutamate increases [84].
Glycerol is an essential ingredient of cell membranes and serves—even more so than the LPR—as a direct marker of cell damage. However, the role of extracellular glycerol in the context of neurointensive care patients with seizures is poorly studied [131]. One of the few studies included just two patients with TBI and electrographic seizures in whom MD detected a higher mean glycerol concentration in comparison with patients who had no seizures. These concentrations, however, were average concentrations during days 1–3 and 7–9 post-injury and not time-locked to the occurrence of seizures [132].
Seizure Activity on Depth Electrode Recordings
Ideally seizure detection would be achieved with sufficient sensitivity using sEEG recordings alone, which as a diagnostic technique carries the advantages of being widely implemented. However, a high percentage of seizures are not detected by sEEG recordings, as suggested by studies in which dEEG and sEEG were recorded simultaneously [80, 85]. In fact, particularly recent advances in the characterization of the physiologic signature of seizures were only possible through the use of dEEG recordings, since a high percentage of seizures detected by dEEG had no visible correlate on sEEG [80, 81, 85, 98].
In a study by Waziri et al. in 14 patients with subarachnoid hemorrhage, 6 patients with seizures on dEEG had no seizure correlate on sEEG [80]. Claassen et al. included 48 patients with subarachnoid hemorrhage in their study. A total of 77 new onset seizures were identified. In this patient sample 38% had seizures on dEEG, whereas only 8% had seizures on sEEG. 43% of seizure events on dEEG recordings had no correlate on sEEG [85]. In their recent publication Vespa et al. reported the combined detection rate for periodic discharges and electrographic seizures in 34 patients with TBI, and found that 43% of seizures and PDs were only visible on dEEG, not on sEEG [81].
Apart from the much higher seizure and PD detection rate, dEEG has the advantage of providing a much higher signal to noise ratio, which opens up a myriad of research options, such as more detailed analyses of seizure onset/offset, spike morphology, and seizure evolution. The greatest limitation of dEEG, however, is its poor spatial resolution in comparison with sEEG. Moreover, despite a justifiable safety profile [79], it is an invasive technique, and insertion, maintenance, and safety control require specifically trained staff. sEEG, on the other hand, provides a global electrophysiological signature of the brain with higher spatial resolution, is non-invasive, can be removed before and replaced after neuroimaging studies, and demands no prior surgical training for electrode placement. To date, despite obvious additional diagnostic information, the use of dEEG is confined to few large academic centers, and continuous sEEG monitoring remains the diagnostic mainstay of seizure detection and monitoring in patients with acute brain injury [68].
Design and reporting of studies on dEEG so far have emphasized advantages of dEEG over sEEG, not focusing on information contained in sEEG which is not reflected in dEEG. The goal of future studies cannot be to replace sEEG by dEEG, but to help define clinical scenarios, in which dEEG constitutes a reasonable diagnostic addition. Moreover, it may be worthwhile exploring sEEG correlates of seizures, primarily detected only on dEEG. Advances in this direction might have a more immediate and direct impact on clinical practice, since continuous sEEG —unlike dEEG—is increasingly available at many centers around the world.
The Clinical Significance of Depth and Surface EEG Seizures
In patients with spontaneous SAH it has been shown that the occurrence of seizures is associated with worse clinical outcome. This holds true in studies in which seizure detection was undertaken using sEEG, and in those studies that used predominantly dEEG. De Marchis et al. studied 402 patients with spontaneous SAH, almost exclusively undergoing sEEG monitoring. 50 (12%) had electrographic seizures on cEEG . Seizures were both qualitatively (presence/absence of seizures) and quantitatively (sum of seizure duration, seizure “burden”) independently associated with worse outcome at 3 months follow up. No significant differences were found between seizure and non-seizure patient groups regarding overall disease severity and other established outcome predictors (Hunt and Hess grade on admission, modified Fisher grade on admission, etc.). Moreover, seizure burden, but not presence of seizures per se, correlated with cognitive performance at 3 months follow up [87].
Claassen et al. included 48 high-grade SAH patients in their study, who were undergoing both sEEG and dEEG. Surprisingly, patients with dEEG-only seizures had worse functional outcome at 3 months than patients with both sEEG and dEEG seizures (50% versus 25% death or severe disability, respectively) [85]. The authors speculate that the failure of seizures to propagate to sufficiently large regions of cortex so as to be seen on sEEG may reflect more severe cortical or subcortical injury.
The question whether seizures on dEEG carry clinical significance and demand treatment is unanswered at this point. It is unclear whether dEEG seizures are an epiphenomenon indicative of severe brain damage (and thus associated with worse outcomes), or if they are causing further brain injury. However, the available evidence on physiologic changes during dEEG seizures with the eventual depletion of energy substrates and brain tissue oxygen, and with the increase of metabolic stress markers, provides good arguments that dEEG seizures are not essentially different from sEEG seizures and therefore should probably be treated [81, 85]. Another study in 479 patients with spontaneous SAH tested the hypothesis of a causal link between post-SAH inflammation and seizures. Patients with seizures compared to patients without seizures on cEEG had higher Systemic Inflammatory Response Syndrome (SIRS) scores. It was furthermore shown that the concentrations of several serum biomarkers of inflammation were higher in patients with seizures. The authors also conducted a mediation analysis showing a potentially causal link between inflammatory response post-SAH and poor long-term outcome mediated by seizures [98].
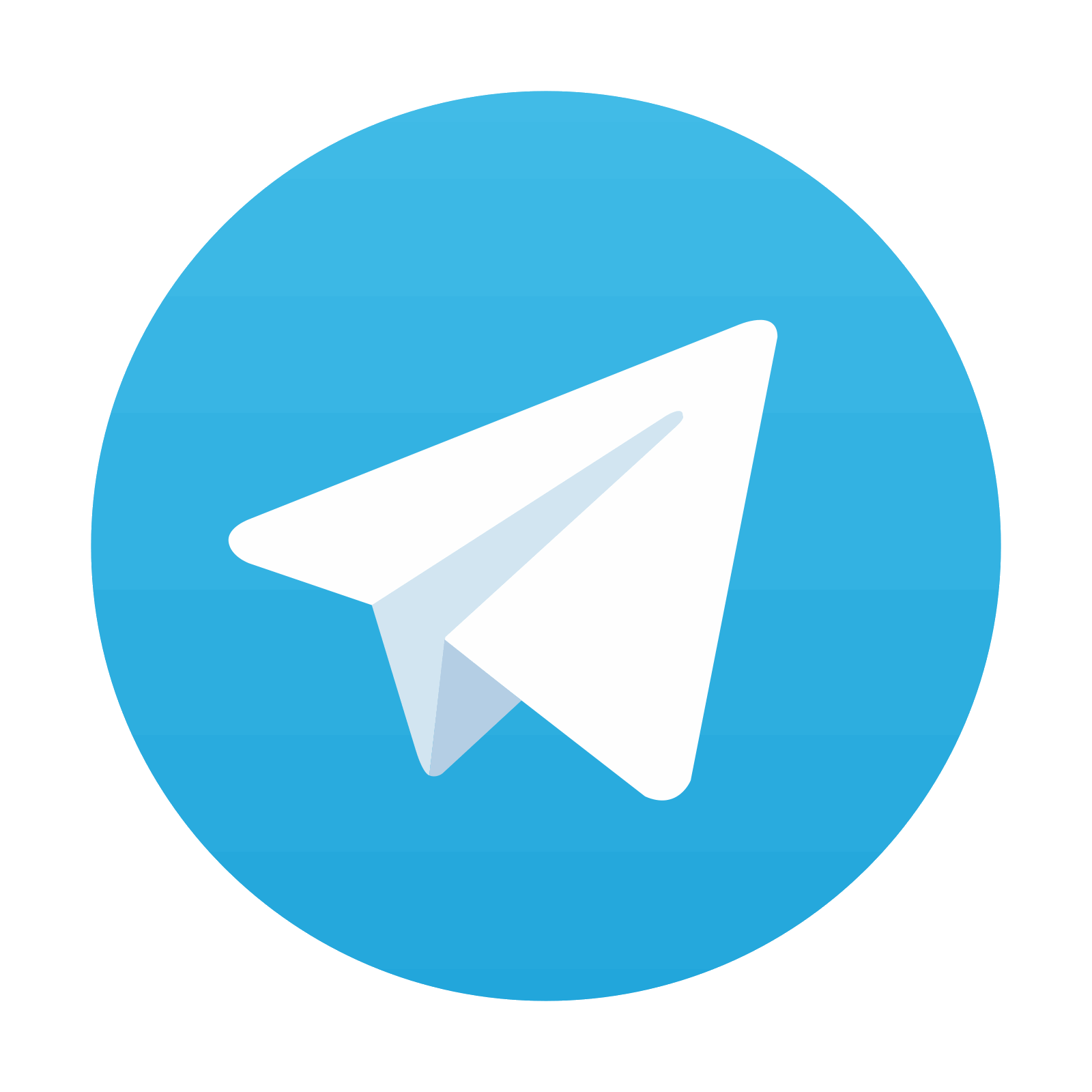
Stay updated, free articles. Join our Telegram channel
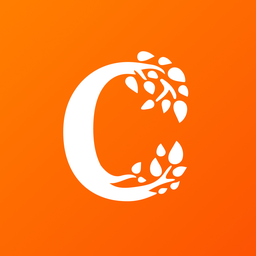
Full access? Get Clinical Tree
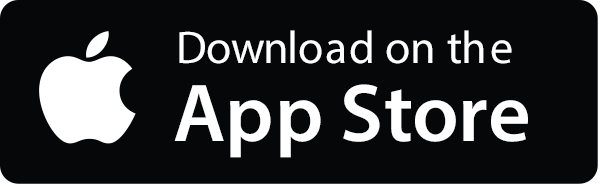
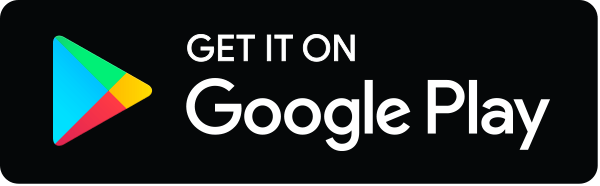