Abstract
Near-infrared spectroscopy (NIRS) is an emerging noninvasive monitoring modality based on chromophore absorption of infrared light. There are four different types of NIRS system—continuous, time domain, frequency domain, and functional—and numerous devices that are now commercially available. These devices differ by the use of different technical components to obtain continuous NIRS values. Their resulting values are not necessarily interchangeable, and few studies have compared them.
Cerebral NIRS is an indirect indicator of perfusion adequacy. Therefore, it allows continuous information on oxygen supply-versus-demand balance. NIRS is indicated when continuous monitoring of cerebral tissue perfusion is considered. Therefore, the application of NIRS covers many procedures including cardiac and noncardiac surgeries but also applications in intensive care and emergency medicine in other clinical and research areas. Furthermore, there is a growing interest in the use of somatic NIRS because both cerebral and somatic desaturation have been associated with worse outcome. The somatic component could serve as an earlier warning of impaired perfusion. There are several limitations in the use of NIRS monitoring and pitfalls that are discussed in detail. As any technology, NIRS is being used as part of a multimodal strategy because abnormal NIRS indicates a perfusion problem but does not provide the precise mechanism. Future studies should explore the impact of this approach on clinical outcome.
Keywords
Brain monitoring, Near-infrared spectroscopy, Ultrasound
Contents
Introduction 180
Physical Principles 181
Types of Near-Infrared Spectroscopy Systems 183
Continuous Wave Near-Infrared Spectroscopy 183
Time Domain Near-Infrared Spectroscopy 185
Frequency Domain Near-Infrared Spectroscopy 186
Functional Near-Infrared Spectroscopy 186
Near-Infrared Spectroscopy Devices 186
INVOS™ 188
FORE-SIGHT™ 189
EQUANOX™ 191
CerOx™ 192
NIRO™ 192
TOS-96™ 193
Regional O3™ System 193
Comparison Between Different Near-Infrared Spectroscopy Machines 194
Comparison of Cerebral Near-Infrared Spectroscopy and Jugular Bulb Oxygen Saturation 195
Differences Between Near-Infrared Spectroscopy and Pulse Oximetry 196
Quantitative and Nonquantitative Near-Infrared Spectroscopy 197
Indications 197
Reading and Interpretation: The Montreal Heart Institute Algorithm 198
Artifactual Brain Desaturation 200
Unilateral Brain Desaturation 200
Bilateral Brain Desaturation 202
Bilateral Isolated Brain Desaturation 202
Bilateral and Regional Brain Desaturation 205
Bilateral and Global Brain Desaturation 207
Other Applications 207
Effects of Anesthetics 208
Advantages of Near-Infrared Spectroscopy 211
Limitations of Near-Infrared Spectroscopy 211
Current Opinion: Role in Various Situations 213
Cardiac Surgery 213
Noncardiac Surgery 215
Intensive Care Unit, Emergency Room, and Cardiology Suite 215
Special Situations 217
Combination Cerebral and Somatic Oximetry 219
Near-Infrared Spectroscopy Simulation and Modeling 220
Conclusion and Future Developments 221
Suggested Readings 221
Abbreviations 221
References 222
Introduction
Cerebral oximetry is a noninvasive monitoring modality based on several physical principles that basically acts as an indirect indicator of perfusion adequacy. Therefore, it allows continuous information on oxygen supply-versus-demand balance. It has numerous applications in the clinical field but also in research.
This monitor is now being evaluated in a variety of different clinical areas, mostly adult and pediatric cardiac surgery, as well as in neurology, neurosurgery, trauma, vascular surgery, during cardiac arrest, and in cardiology procedures.
Despite various uses in many clinical settings and numerous physiological applications, near-infrared spectroscopy (NIRS) has been used mainly to detect and correct intraoperative cerebral desaturations in cardiac surgery. The prognostic value of these desaturations, the specific thresholds requiring intervention, and the clinical impact of this type of monitoring are still under current investigation.
In this chapter, we will review the underlying physical principles on which cerebral oximetry is based. The indications, current applications, and limitations of this monitoring modality will be reviewed. There is an abundant literature on NIRS with more than 17,323 articles on PubMed. We will not be able to review the entire literature on cerebral oximetry; however, we will report systematic reviews when available. In addition, we will share our experience using this monitoring modality at the Montreal Heart Institute since 2002.
Physical Principles
NIRS is a method for noninvasive monitoring of tissue oxygenation and hemodynamics. The unique ability of light in the near-infrared (NIR) range (700–1000 nm) to detect the oxygenation state of living tissue was first realized by Jobsis in 1977. Jobsis described a spectroscopic technique to determine the oxidation status of cytochrome aa 3 in intact cat brain and rat heart using light transmission through the tissue in a way similar to using oximetry for hemoglobin saturation. Since then, NIRS has evolved as a technique for in vivo and continuous monitoring of cerebral and tissue oxygenation.
In a nonscattering medium, the attenuation of light due to absorption is a measure of the concentration of chromophores present. The relation between absorption and the concentration of the chromophores is described by the Lambert–Beer law. This law states that attenuation is directly proportional to three variables: the chromophore concentration, the distance traveled by the light between the source and detector, and the absorption coefficient of the chromophore, which describes the absorptive properties of a chromophore at a given wavelength.
Attenuation of light in a scattering medium such as biological tissue is a complex function of scattering, absorption, and reflection, as well as the geometry of the tissue and measurement optics. Absorption and scatter in tissue depend on wavelength. However, reflection is a function of the angle of the light beam and the tissue surface.
NIRS is based on several physical characteristics of NIR light. First, the skin, scalp, and skull are relatively transparent to NIR light, allowing transmission of NIR photons into the brain. Second, as these photons pass through the brain, they undergo attenuation as a result of scattering and absorption by specific chromophores. These chromophores are intravascular hemoglobin and intracellular cytochrome aa 3. Finally, measurement of changes in cerebral chromophore concentration is related to the overall optical attenuation of NIRS light by a modified Beer–Lambert Law.
A = ( a [ c ] LB ) + G
The attenuation due to scattering and the geometric factor is not known; thus the concentration of chromophore cannot be quantified absolutely. Nonetheless, in a given tissue with a fixed optical geometry, it is likely to remain constant. Changes in attenuation, therefore, can be attributed solely to changes in the concentration of chromophores.
Δ [ c ] = Δ A / ( a × L × B )
Optical densities are measured at several wavelengths selected for their proximity to absorption peaks of chromophores of interest. NIRS measures changes in concentration of oxyhemoglobin (HbO 2 ), deoxygenated hemoglobin (HHb), and from their sum, changes of total hemoglobin (THb), as well as changes in the concentration of oxidized cytochrome aa 3 (CytOx). In addition, claims are made to measure the ratio of HbO 2 /THb, and this is reported as “regional oxygen saturation” (rSO 2 ), as “tissue oxygen index” (TOI), or as “cerebral oxygen tissue saturation” (SctO 2 ).
Oxyhemoglobin and deoxygenated hemoglobin have well-defined absorption peaks at 758 and 929 nm, respectively; while at 798 nm the absorption factors of these two chromophores are equal ( Fig. 7.1 ). CytOx shows a broad absorption peak with a maximum at 830 nm and the absorption spectrum of deoxidized cytochrome aa 3 is completely flat. Therefore, the measurement of the oxygenation status of hemoglobin can be performed in a relatively simple fashion, whereas the assessment of cytochrome aa 3 oxidation requires elaborate algorithms and use of multiple wavelengths.

Cytochrome oxidase is the terminal member of the mitochondrial respiratory chain, producing the energy necessary for conversion of adenosine diphosphate (ADP) to adenosine triphosphate (ATP). If oxygen is unavailable, electron transfer cannot take place, and electrons accumulate on the haem and copper atoms of cytochrome aa 3, causing it to become reduced. When oxygen becomes available, electrons are transferred to oxygen, and cytochrome aa 3 becomes oxidized. Thus, monitoring changes in the oxidized form of the enzyme cytochrome aa 3 can provide valuable information on oxidation-reduction state of the enzyme and hence on oxygenation at the cellular level.
Types of Near-Infrared Spectroscopy Systems
The various types of NIRS system are summarized in Table 7.1 .
|
Continuous Wave Near-Infrared Spectroscopy
The continuous wave NIRS (CW NIRS) is the type of measurement made in the earliest clinical NIRS studies. The measured attenuation (A) at the receiving optode cannot be quantified in CW NIRS, as it contains an unknown light loss due to tissue scattering, increased path length, and thereby increased absorption. It detects changes of attenuation of the detected light from some arbitrary start time. CW NIRS can only be used as a trend oxygenation monitor.
These instruments generally employ either a multiple discrete wavelength source or a filtered white light source, and they measure the light attenuation using either a photomultiplier, photodiode, or an avalanche photodiode detector. CW NIRS systems are less expensive and have faster sampling rates than time-resolved and frequency domain NIRS. Second derivative spectroscopy and spatially resolved spectroscopy are examples of using CW NIRS as concentration monitors.
Second Derivative Near-Infrared Spectroscopy
It has been suggested that CW NIRS could also provide estimates of the differential path length factor and thereby quantifies the measured changes in concentration. In the near-infrared range, the water spectrum contains three major features, a prominent peak centered around 965 nm and two other small peaks around 820 and 740 nm. The 965 peak is large enough to allow accurate curve-fitting of its shape to the known spectrum of water.
The concentration of water in the brain is usually known to be within 2%–3%; therefore, it is possible to estimate the DPF at 965 nm using the ratio of the peak amplitudes and the known interoptode spacing.
The principle of this technique is that if the measured spectrum sits on an unknown constant attenuation, then this can be removed by taking the first derivative of the spectrum with respect to wavelength. If there is an additional unknown, but linearly wavelength-dependent, attenuation present, this will appear as a constant in the first derivative, which can be removed by taking the second derivative. This should leave a flat spectrum containing only features corresponding to the second derivative of the absorption spectra of any chromophores in the tissue. In the NIR, the second derivative of the water spectrum has three components corresponding to the previously mentioned features. Hemoglobin (Hb) has a large feature corresponding to the 760-nm absorption peak, but HbO 2 and CytOx have negligible features. Therefore, it is possible to calculate the ratio of the Hb concentration to the water concentration and thereby obtain an absolute measure of changes in the Hb concentration.
Spatially Resolved Near-Infrared Spectroscopy
Spatially resolved spectroscopy (SRS) is one of the most used NIRS systems. The SRS system incorporates several detectors housed in a single probe, with an interoptode distance of 4–5 cm ( Fig. 7.2 ).

The combination of the multidistance measurements of optical attenuation allows calculation of the relative concentrations of HbO 2 and HHb in the illuminated tissue. This calculation is derived from the relative absorption coefficients obtained from the slope of light attenuation at different wavelengths over a distance measured at several focal points from the light emission.
Time Domain Near-Infrared Spectroscopy
Time domain spectrometers are also called time-of-flight or time-resolved systems. They generally employ a semiconductor or solid-state laser to generate ultra-short pulses. Such a system measures the attenuation by either a synchroscan streak camera or a time-correlated single photon counting in which a photon counting detector detects and sorts the received photons by their time of arrival. Time domain spectrometers are the most accurate spectrometers in separating absorption and scattering effects. However, such spectrometers are not for routine use because of the sampling rate, the instrument size, the instrument weight, the necessity for cooling, the lack of stabilization, and the cost.
Frequency Domain Near-Infrared Spectroscopy
Frequency domain spectrometers are also called frequency-resolved or intensity-modulated systems. The radio frequency–modulated light cannot exceed 200 MHz. They generally employ a laser diode, or modulated white light sources. They measure the attenuation, phase shift, and modulation depth of the exiting light by either a photon-counting detector or gain-modulated area detector. Tissue scattering and absorption can be computed from frequency and phase shift.
The frequency domain spectrometers perform measurements of changes in intensity, phase, and modulation by using either (1) single wavelength and a fixed interoptode distance, (2) multiple wavelengths and a fixed interoptode distance, (3) or single wavelength and multiple interoptode distances.
Functional Near-Infrared Spectroscopy
It appeared after the development of multichannel CW NIRS imaging systems, which allow the generation of images of a larger area of the subject’s head with high temporal resolution, and thereby the production of maps of cortical oxygenation changes. It is known as either functional NIRS (fNIRS), diffuse optical imaging (DOI), Diffuse optical Tomography (DOT), or near-infrared imaging (NIRI). It requires sophisticated image reconstruction algorithms to convert the transmittance measurements into 3D images and is far from clinical use due to high cost.
Near-Infrared Spectroscopy Devices
A NIRS device exists out of a light source (emitting optode) to deliver light to the tissues at a known intensity and at two (or more) wavelengths surrounding the isobestic point, and a light detector (receiving optode), which measures the intensity of the exiting light. A computer translates the change in light intensity to clinical useful information ( Figs. 7.3 and 7.4 ).


The position of the receiving optode from the light source can be either contralateral (transmission NIRS), which is most frequently used in infants, or ipsilateral (reflectance-mode NIRS). In addition, if two receiving optodes are used such as in SRS and NIRS, it is referred to as multidistance. Multidistance NIRS approach allows one to differentiate between light attenuation caused by skull and overlying tissues and light attenuation caused by cerebral tissue.
For cerebral oximetry, self-adhesive emitter/sensor pads applied to the skin of the forehead measure light attenuation noninvasively at a set distance from a NIRS-emitting light source. The absorption of the emitted light is directly proportional to chromophore concentration, the absorption coefficient of the chromophore, and the distance that the light travels between the emitting source and the detector, as stated in the Beer–Lambert law.
Oxyhemoglobin and deoxyhemoglobin have different and characteristic peak absorptions in the near-infrared spectrum, but both absorb light at an isobestic wavelength around 800 nm. The absorption of light at the isobestic wavelength allows for the measurement of total hemoglobin concentration. Thus, using a modification of the Beer–Lambert law, NIRS provides a measurement of the concentration of oxygenated hemoglobin in relation to total hemoglobin concentration.
Today, several NIRS devices are available ( Tables 7.2 and 7.3 ), and all have similar technology but with differences in the number and absolute value of wavelengths, in the computational algorithms, in the source of light, and in the distances between light emitted. The INVOS™, FORE-SIGHT™, EQUANOX™, and CerOx™ devices and the O3™ Regional Oximeter System are approved by Food and Drug Administration and Health Canada.
|
Company | Device | Variables | Wavelengths (nm) | Type |
---|---|---|---|---|
CAS Medical | FORE-SIGHT™ ELITE, (CAS Medical Systems, Branford, CN) | Cerebral and somatic tissue oxygen saturations | 690, 778, 800, 850 nm | Saturation (absolute) |
COVIDIEN | INVOS™ 5100, (Somanetics/Covidien, Inc., Boulder, CO) | Cerebral and somatic tissue oxygen saturation | 730, 810 nm | Saturation |
Hamamatsu Photonics | NIRO™ 300, (Hamamatsu Photonics, Hamamatsu City, Japan) | Cerebral (HbO 2, HHb, CytOx, TOI) | 775, 825, 850, 905 nm | Concentration and saturation |
Masimo | O3™ (Masimo, Irvine, CA) | Cerebral oxygen saturation | 730, 760, 805, 880 nm | Saturation (absolute) |
Nonin Medical | EQUANOX Advance™ 8004CA, (Nonin Medical Inc., Plymouth, MN) | Cerebral and somatic tissue oxygen saturations | 730, 760, 810, 880 nm | Saturation |
Ornim Medical | CerOx™, (Ornim Medical, Lod, Israel) | Cerebral and somatic blood flow and saturation | Ultrasound-tagged near-infrared spectroscopy (UT-NIRS), and three wavelengths between 780 and 830 nm | Saturation and blood flow |
TOSTEC Co. | TOS-96™, (TOSTEC Co., Tokyo, Japan) | Cerebral rSO 2 | 750, 850, 810 nm | Saturation |
INVOS™
The INVOS™ system utilizes near-infrared light at wavelengths that are absorbed by hemoglobin (730 and 810 nm). It has two receivers at different distances from the light emitter to correct for surface tissue overlying the brain ( Fig. 7.5A ).

Sensors for light detection are placed 30 and 40 mm from the light-emitting diode. For INVOS™ the assumed arterial:venous ratio is 25%/75%, upon which the oximetry values are calculated. Light travels from the sensor’s light-emitting diode to either a proximal or distal detector, permitting separate data processing of shallow and deep optical signals. INVOS™ system’s ability to localize the area of measurement, called spatial resolution, has been empirically validated in human subjects. Data from the scalp and surface tissue are subtracted and suppressed, reflecting rSO 2 in deeper tissues. A single absolute value, rSO 2 , is calculated by an algorithm, which is thought to be independent of path length. The same concept applies to somatic monitoring.
FORE-SIGHT™
On the FORE-SIGHT™ monitor, the sensors are 15 and 50 mm from the laser-generated light source. It uses laser-emitting diodes to generate light at four different wavelengths (690, 778, 800, and 850 nm), and “ wavelength”-resolved spectroscopy, with two detectors. The scalp detector samples returning light from the extracranial tissue, whereas the brain detector samples returning light signal from both the brain and the extracranial tissue ( Fig. 7.5A ). The signal from the scalp detector is used to cancel extracerebral interference from the signal of the brain detector to obtain information that mostly comes from the brain.
The SctO 2 is determined from the ratio ((HbO 2 )/(HbO 2 + HHb)) × 100%, which assumes a mixture of venous to arterial to venous blood of 70/30, respectively. It is claimed the monitor measures absolute values that indicate a patient’s cerebral tissue oxygen saturation status.
EQUANOX™
EQUANOX™ utilizes four wavelengths (730, 760, 810, and 880 nm) of light to measure the balance of oxy- and deoxyhemoglobin, while compensating for tissue factors that reduce rSO 2 accuracy. It utilizes a dual light-emitting and -detecting sensor architecture, which has been shown to more effectively target the cerebral cortex, eliminating surface artifacts that interfere with measurement accuracy.
The EQUANOX™ Classic 7600 monitor system uses two separate sets of light-emitting diodes and two light sensors that are 20 and 40 mm from the light source ( Fig. 7.5B ). It uses a more complex analysis process whereby the 40-mm detector for one light source serves as the proximal sensor for the other device.
The EQUANOX™ Advance series cerebral/somatic oximetry system effectively isolates targeted tissue and automatically considers the light attenuation changes caused by myelination variation. Also, age, skin color, and hematocrit did not affect these values.
CerOx™
The CerOx™ monitor (Ornim Medical) uses a laser light source and has one sensor. This method allows the light-penetration profile to be assessed, thereby enabling the light to be focused on the desired depth and volume of brain tissue. The monitor employs a new technology, ultrasound-tagged near-infrared spectroscopy (UT-NIRS), leveraging the effect of ultrasound waves on light to tag it. This technology uses the ability of near-infrared light to measure regional oxygen saturation in combination with ultrasound that can achieve localization via the acousto-optic effect. Monitoring of the reflected ultrasound provides an additional indicator of local tissue cerebral blood flow. It uses NIRS and ultrasound to assess cerebral oxygenation and blood flow in a noninvasive manner.
The probe emits light at three wavelengths between 780 and 830 nm and detects the scattered light at a distance of 12 mm from the emitter. Low-power ultrasound waves are also emitted to induce the UT-NIRS signal by modulating light via the acousto-optic effect. A spectral analysis is then performed to calculate the saturation of hemoglobin within the region of interest.
NIRO™
The original NIRO-500™ measured only changes in concentrations from an arbitrary point using the modified Beer–Lambert law (MBL). Another clinical tissue oxygenation monitor has been developed, the NIRO-300™, which measures the concentration changes (ΔHbO 2 , ΔHHb, ΔCytOx) by MBL and a TOI by SRS ( Fig. 7.3 ). The following variables are monitored by NIRO-300™:
- 1.
oxygenated hemoglobin (ΔHbO 2 ),
- 2.
deoxygenated hemoglobin (ΔHHb),
- 3.
total hemoglobin (ΔTHb) = ΔHbO 2 + ΔHHb,
- 4.
oxidized cytochrome aa 3 (ΔCytOx).
- 5.
tissue oxygen index (TOI) = (HbO 2 /THb) × 100
Measurements of ΔHbO 2 , ΔHHb, and ΔCytOx start at zero level because the absolute values of these parameters are not known. However, TOI is reported as an absolute value. This is attributed to the use of SRS, which incorporates several detectors housed in a single probe placed 4–5 cm from the source ( Fig. 7.5B ). It uses four wavelengths: 775, 825, 850, and 905 nm. Combination of these multidistance measurements of optical attenuation with the usual multiwavelength spectroscopy data allows calculation of the relative concentrations of HHb and HbO 2 in the illuminated tissue and therefore an estimate of the mean tissue hemoglobin saturation even though the component HbO 2 can be reported only as changes.
NIRO-200NX™ is a new tissue (cerebral and somatic) oxygen monitor machine introduced by Hamamatsu. It measures TOI and normalized tissue hemoglobin index (nTHI), showing the percentage change in the amount of initial hemoglobin, as well as ΔHbO 2 , ΔHHb, and ΔTHb, all in real time. The probe emits light at three wavelengths: 735, 810, and 850 nm.
TOS-96™
TOS-96™ (TOSTEC Co., Tokyo, Japan) is attached to the patient’s forehead for monitoring the rSO 2 in the bilateral frontal lobes at a depth of approximately 3 cm beneath the skin. Each sensor consists of one silicon diode that receives the signal and three laser-emitting diodes (LEDs) that emit near-infrared light of wavelengths 750, 850, and 810 nm, for measurement of HHB, HbO 2 , and Hb level, respectively. The rSO 2 % is calculated from the NIRS data, using the following equation :
rSO 2 = ( oxyHb ) / ( oxyHb + deoxyHb ) × 100
Regional O3™ System
The O3™ Regional Oximeter System (O3™ System) (Masimo Corporation, Irvine CA), which consists of the O3 Module and O3™ Sensor, is a patient-connected, LED-based technology, noninvasive oximeter designed to continuously measure and monitor rSO 2 . O3™ Regional Oximetry is intended for use as an adjunct monitor of absolute and trended rSO 2 of blood in the cerebral region under the sensors. The O3™ System’s operating principle is based on multidistance diffuse reflectance NIRS and consists of one light emitter and two photodetectors. It uses four wavelengths of light to examine a cross-section tissue microvasculature (a mixed bed of arterioles, capillaries, and venules) and analyzes the received light for calculating deep-tissue oxygen saturation. For the purpose of comparison against a standard reference, blood in the cerebral tissue is approximated as a mixture of venous (70%) and arterial (30%) blood. The main advantage of this technology is that the information is displayed simultaneously on the monitor with concurrent bilateral SedLine, pulse oximetry, capnography, and oxygen reserve index (ORI). SedLine Sedation Monitor is a patient- connected, 4-channel processed electroencephalogram (EEG) monitor designed specifically for intraoperative or intensive care unit (ICU) use. It displays electrode status, EEG waveforms, density spectral array (DSA), and patient state index (PSI). The ORI is a novel pulse oximeter–based nondimensional index that ranges from 1 to 0 as PaO 2 decreases from about 200 to 80 mm Hg and is measured by optically detecting changes in SvO 2 after SaO 2 saturates to the maximum. The O3™ System was validated by Redford et al. in 27 healthy volunteers undergoing controlled hypoxia.
Comparison Between Different Near-Infrared Spectroscopy Machines
Several studies compared readings obtained from different NIRS devices in various clinical situations, but the results are not encouraging. Hessel et al. found an oxygen level–dependent difference between INVOS™ 5100C and FORE-SIGHT™ cerebral oximeters in 12 term newborn infants. Their readings differed as much as 20% at the lowest range of saturations. Others compared INVOS™ 5100C and EQUANOX™ 7600 cerebral oximeters during cardiac surgery and found that both devices are not interchangeable with a mean bias of −5.1% and limits of agreement of ±16.37% when comparing absolute values. Dix et al. compared five sensors of three commonly used devices INVOS™ (INVOS neonatal, INVOS pediatric and INVOS adult sensors), FORE-SIGHT (neonatal sensor), and EQUANOX (adult sensor) in preterm neonates. They found good correlation between different NIRS sensors. However, substantial differences between absolute cerebral oxygenation values measured with the adult sensor and the pediatric and neonatal sensors range between 10% and 14%, being higher in the pediatric and neonatal sensors.
Interestingly, Bickler et al. studied factors that affect the performance of five cerebral oximeters (EQUANOX 7600 in 3- and 4-wavelength versions, FORE-SIGHT, INVOS TM 5100C, and the NIRO-200NX) during stable isocapnic hypoxia in volunteers. Six steady states of arterial oxygen saturation (SaO 2 ) levels between 100% and 70% were achieved by changing inspired oxygen (FIO 2 ), while end-tidal carbon dioxide (ETCO 2 ) was maintained constant. At each plateau, simultaneous blood samples from the jugular bulb and radial artery were analyzed with a hemoximeter. Each cerebral oximeter’s bias was calculated as the difference between the instrument’s reading (cerebral saturation) with the weighted saturation of venous and arterial blood, as specified by each manufacturer (INVOS: 25% arterial/75% venous; FORE-SIGHT, EQUANOX, and NIRO: 30% arterial/70% venous). A calculated bias was observed between the oximeters readings and the weighted values of arterial and cerebral mixed venous oxygen saturation. FORE-SIGHT™, NIRO-200NX™, and EQUANOX (3 wavelength version) have significant positive mean bias during hypoxia. In addition, there was significant variation between-subject and between-instrument with substantial variably in baseline readings between the five instruments.
Such results indicate that absolute cerebral oxygenation values cannot be provided by the five devices, and the absolute threshold of saturation that results in tissue damage is difficult to be determined. It is clear that measurements were not quite equivalent and varied depending on the chosen NIRS device, and different NIRS devices provide us with different values. In addition, there were significant differences in absolute values and dynamic measurements between different devices, and there was a large intra- and interindividual variability and a variable repeatability.
In a multicenter trial conducted by Deschamps et al., three devices were used. Although the goal of the study was not to compare them, the number of decrease in rSO 2 below 20% with the different NIRS devices was 1.5, 1.7, and 2.3 desaturations/patient recruited with the FORE-SIGHT, EQUANOX Classic 7600, and INVOS 5100C-PB, respectively. A systematic review of cerebral oxygenation devices was published in 2014 by Douds et al. Most of the studies, so far, have been done with the INVOS.
Such differences could be explained by the following: First the differences in technical aspects such as different algorithms, near-infrared light emission source, number of wavelengths, or scattering subtraction may be underlying causes. Two, since no real reference value exists for rSO 2 , it is not possible to state if one monitor is more valid than another. Finally, the accuracy of quantitative data by NIRS is limited by inaccuracies in the estimation of optical path length for light transmitted through tissue. Until real-time path length measurements are incorporated, relative changes will form the basis of NIRS.
Comparison of Cerebral Near-Infrared Spectroscopy and Jugular Bulb Oxygen Saturation
Since cerebral NIRS and jugular bulb oxygen saturation (SjO 2 ) both aim to reflect cerebral oxygenation, it is important to see how well the two correlate ( Table 7.4 ). Although SjO 2 and rSO 2 are known to be similar in some studies, the limits of agreement are wide in others. Also, there was no clear consistent agreement between SjO 2 saturation and rSO 2 measured by different NIRS machines. However, narrow limits of agreement appeared when considering rSO 2 as 70%–75% venous, and 25%–30% arterial. This could be explained by the global nature of SjO 2 , versus the small region of brain tissue reflected in rSO 2 , by extracranial contamination of the NIRS signal and lack of gold standard for NIRS measurements. Such results showed that the two methods are not interchangeable. Which one is “right” remains a subject of controversy, since both methods probably measure different entities and/or each monitor offers a different window for brain monitoring.
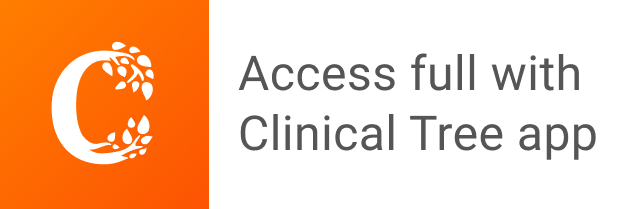