Neurodegenerative diseases, of which Alzheimer disease and Parkinson disease are the most common, are a large and diverse group of neurologic disorders characterized by death of neurons.
The major processes by which neurons die are necrosis and apoptosis (programmed cell death); both processes involve cascades of proteins including proteolytic enzymes of the caspase family. These mechanisms, once thought entirely distinct, have significant overlap.
Processes that can initiate cell death include mitochondrial dysfunction, excitotoxicity, oxidative stress, and abnormal protein aggregation.
Abnormal mitochondrial function plays a key role in apoptosis and other forms of neurodegeneration.
Excitotoxicity involves excessive activation of neurons via stimulation of glutamate receptors or other mechanisms of depolarization, which trigger biochemical cascades—many of them secondary to excessive Ca2+ flux into neurons—that lead to cell death.
The pathologic hallmarks of Alzheimer disease, the most common cause of severe memory impairment in the elderly, are senile plaques, neurofibrillary tangles, dystrophic neurites, and neuronal loss.
The development of Alzheimer disease may be due to the improper biochemical processing of amyloid precursor protein (APP) and the subsequent accumulation of β amyloid. A role for the microtubule-associated protein tau has also been implicated.
Inherited forms of Alzheimer disease have been linked to mutations in APP or in proteins called presenilins, which regulate the processing of APP and several other proteins.
Currently there is no effective treatment for Alzheimer disease, although several pharmacologic strategies for preventing the buildup of β amyloid, promoting its clearance, or counteracting its deleterious effects appear promising.
Frontotemporal dementias comprise several other neurodegenerative disorders, roughly half of which involve abnormal aggregation of tau.
Loss of dopamine input to the basal ganglia causes the symptoms of Parkinson disease; restoration of dopamine function, for example, with the dopamine precursor L-dopa, remains the mainstay of therapy for the disease.
Rare familial forms of Parkinson disease are caused by mutation in several genes related to protein aggregation, including α-synuclein and parkin.
Huntington disease is caused by a mutation in the gene for huntingtin; the mutation increases the number of glutamine residues expressed in the protein, which is pathogenic.
Amyotrophic lateral sclerosis (ALS) is caused by the selective death of upper motor neurons in the cerebral cortex and/or lower motor neurons in the spinal cord.
In general terms, cell death occurs by either of two distinct processes, termed necrosis and apoptosis, which are characterized by very different histologic and biochemical signatures. In necrosis, the stimulus that triggers death is itself often the direct cause of the demise of the cell. In apoptosis, by contrast, the death stimulus activates a cascade of events that orchestrate the destruction of the cell. For this reason, apoptosis is referred to as programmed cell death. Unlike necrosis, which is a pathologic process, apoptosis is part of normal development; however, it also occurs in a variety of diseases.
Because the vast majority of neurons in an adult are postmitotic, the brain has limited capacity to compensate for cells lost during pathologic processes, such as neurodegeneration, stroke, and traumatic injury. As a result, enormous attention has been focused on understanding mechanisms of neuronal cell death in the hope that novel therapeutics can be developed to minimize or prevent cell loss. These efforts have focused on necrotic and apoptotic processes, although recent findings have revealed partly overlapping molecular pathways underlying the two. Identification of multiple forms of nonapoptotic programmed cell death has further blurred the distinction between necrosis and apoptosis. In addition, two forms of cell death appear to be unique to neurons: excitotoxicity and death induced by protein aggregation. This chapter begins with a basic overview of the molecular and cellular mechanisms of these distinct processes of neurodegeneration. The second portion of the chapter focuses on several severe brain disorders, including Alzheimer disease, frontotemporal dementia (FTD), Parkinson disease, Huntington disease, and amyotrophic lateral sclerosis (ALS), each of which is characterized by a unique pattern and mechanism of neurodegeneration. Although disease-altering treatments for these disorders remain lacking, we will discuss new approaches to intervene therapeutically based on our evolving knowledge of the molecular mechanisms of disease pathogenesis.
Necrotic cell death in the central nervous system (CNS) occurs in response to several types of environmental insults, often termed “cellular stress.” Examples of cellular stresses include ischemia (lack of oxygen), UV irradiation, virus infection, free radicals, and various toxins. Necrosis occurs preferentially in areas of the CNS that are most severely affected by abrupt biochemical collapse, which leads to the generation of free radicals and excitotoxins. The histologic features of necrotic cell death are mitochondrial and nuclear swelling, dissolution of organelles, and condensation of chromatin around the nucleus.
Intracellular pathways activated by these cellular stresses are designed to turn off nonessential cellular functions, limit the internal damage, activate repair mechanisms, and allow the cell to survive homeostatic challenges. However, when a neuron receives excessive injury, stress response pathways are overwhelmed, resulting in depletion of energy stores and loss of membrane integrity. The resulting necrotic neurons and the organelles they contain swell until their membranes eventually burst. Necrosis is exactly what might be expected of a cell undergoing failure of active transport, membrane integrity, and osmotic stability, all of which ultimately result in disintegration. Necrosis is accompanied by the leakage of cytoplasmic and organelle contents into the extracellular space; such leakage can be toxic to neighboring cells and can promote inflammatory processes. The molecular mechanisms underlying responses to cellular stress are becoming increasingly well understood 18–1. Given these mechanisms, and the rapidity with which the process occurs, necrotic cell death is extremely difficult to treat or prevent.
18–1 Cell Death and Survival Pathways
Tremendous progress has been made in understanding the dynamic balance between death signals (orange in figure) and survival signals (purple in figure) in neurons. The figure depicts just a small portion of the complex intracellular signaling pathways governing cell death and survival. There is considerable overlap between mechanisms of necrosis and apoptosis, such that many of the pathways shown in the figure contribute to both processes.
Cell death can be triggered by intracellular or extracellular pathways. The extracellular pathway involves transmembrane receptors, such as Fas (the name comes from an antibody screen), tumor necrosis factor-α (TNF-α), and p75 (also called the low-affinity neurotrophin receptor; see Chapter 8). These proteins contain a conserved death domain, which recruits and activates caspases (Casp) 8 or 10 by means of death-domain adaptor proteins such as TNF receptor–associated death domain (TRADD) and Fas-associated death domain (FADD). These caspases, in turn, activate effector caspases, caspases-3, -6, and -7, which trigger cell death via the fragmentation of cell structures.
In contrast to the extracellular pathway, the intracellular pathway relies on permeabilization of the outer membrane of mitochondria by pro-death Bcl-2 family members. Proteins such as Bcl-2 associated protein X (Bax), Bcl-2 homologous antagonist/killer (Bak), or Bad oligomerize in the mitochondrial membrane and permit the release of pro-death proteins such as cytochrome c (Cyt c), second mitochondrial activator of caspases (SMAC), endonuclease G (Endo G), and apoptosis inducing factor (AIF). Prosurvival Bcl-2 family members, including Bcl-2 and Bcl-Xl, interfere with Bax and inhibit mitochondrial permeabilization. On release from the mitochondria, Cyt c binds to a cytoplasmic adapter protein called apoptosis-activating factor 1 (Apaf1) that allows for oligomerization and autoproteolytic activation of caspase-9. Activated caspase-9, much like caspase-8, can cleave caspases-3, -6, and -7 to initiate a self-amplifying cascade that leads to apoptosis. SMAC induces apoptosis by inhibiting the prosurvival inhibitors of apoptosis (IAPs) that inhibit caspases. Endo G and AIF promote apoptosis by translocating to the nucleus where they induce large-scale fragmentation of DNA.
Cellular stress, which can be produced by a variety of agents and environmental factors, induces deleterious effects on cells in part via activation of Jun N-terminal kinase (JNK) and p38, both types of stress-activated protein kinase (SAP kinase). JNK and p38 are protein serine–threonine kinases that are involved in one branch of the MAP kinase cascades (see Chapter 4 for more detailed discussion of these cascades). Activation of specific forms of MAP kinase kinases (MEK kinases), a process that depends on the Ras-like small G protein Rac, leads to activation of a series of MEKs and ultimately to the activation of JNK or p38. In vitro and in vivo studies have indicated that JNK and p38 are involved in both necrotic and apoptotic forms of cell death. A primary target of JNK is the activation of transcription factors c-Jun and activating transcription factor 2 (ATF2). c-Jun levels are elevated in the CNS after cellular stress, and dominant negative forms of c-Jun protect cells from certain forms of injury.
An interesting question raised by these findings is the following: what is the normal function of cellular stress pathways? Presumably, transient activation of JNK and p38 triggered by more mild forms of stress serves to protect the cell from long-term injury by initiating homeostatic responses. Perhaps excessive or more sustained activation of these pathways, as seen under abnormal conditions, leads to cell death. Further work is needed to better understand the normal functioning of these pathways and to assess whether their pharmacologic inhibition may be of use under certain pathophysiologic conditions.
One of the prototypical triggers for apoptosis, at least in vitro, is the withdrawal of neurotrophic factors. Neurotrophic factor receptors, such as the TrkA receptor for NGF or the IGF-1 receptor for insulin-like growth factor, activate prosurvival signaling cascades; these involve the sequential activation of insulin receptor substrate (IRS), phosphatidylinositol-3-kinase (PI3K), and AKT (a serine/threonine kinase). AKT phosphorylates proapoptotic proteins such as Bad and caspase-9, inhibiting their apoptotic action.
Apoptosis, a process of programmed cell death as noted above, is essential for the survival of an organism. In the nervous system, it enables the pruning of excess neurons during development, and thereby contributes to the formation of anatomically precise and correct connections between nerve cells. However, increasing evidence indicates that apoptotic mechanisms of neuronal death also contribute to several neurodegenerative disorders and to decrements in brain function associated with aging. Consequently, considerable attention is focused on understanding the precise molecular basis of apoptosis and on developing medicinal agents that interfere with these processes. A brief overview of the molecular pathways of apoptosis is provided in 18–1.
Apoptotic neurons undergo a series of tightly regulated changes characterized by chromatin aggregation, exocytosis of membrane-bound cytoplasmic fragments (apoptotic bodies), and progressive loss of cell volume. Apoptosis is generally recognized as a series of steps undertaken by a doomed cell in order to prevent necrosis. In contrast to necrosis, during apoptosis potentially dangerous intracellular material is packaged into vesicles that can be safely taken up into surrounding cells by endocytosis and metabolized. Unlike necrosis, which is a passive process, cell death by apoptosis results from the execution of active and well-regulated genetic programs. In many biologic systems, inhibition of gene transcription or protein synthesis can prevent apoptosis, underscoring its active nature.
Apoptosis may result in the death of neurons only moderately affected by injury. For instance, it may affect neurons that undergo a mild or short-lived ischemic event, such as those in a peri-infarct area, which is the area between severely ischemic and normal tissue in the period immediately following a stroke. In cortical cell cultures treated with very high levels of N-methyl-D-aspartate (NMDA) (which activates NMDA glutamate receptors; Chapter 5) or nitric oxide (NO) plus superoxide, neuronal death is generally observed to be necrotic; however, with lower levels of NMDA or NO–superoxide, apoptosis occurs. These findings suggest that severely damaged neurons undergo necrosis before a more controlled process of apoptosis can occur, whereas in moderately damaged neurons apoptotic processes may be initiated as a way to dispose of damaged neurons in a safer manner.
Thus, apoptosis provides a safe means of removing damaged cells, including those that are potentially cancerous. For example, the tumor suppressor p53 is a transcription factor that activates proapoptotic gene expression in response to DNA damage that might lead to cell transformation (see figure in 18–1). For this reason, as the field strives to develop inhibitors of apoptosis to limit neuronal loss in neurodegenerative disorders, caution must be exercised with respect to potentially serious side effects of inhibiting this crucial physiologic process.
One of the hallmark characteristics of apoptosis involves the breakdown of double-stranded DNA into nucleosomal segments (Chapter 4). This breakdown makes it possible for apoptosis to be observed, because the resulting segments are equally spaced and can be visualized as DNA “laddering” on an electrophoretic gel. DNA laddering can also be visualized in tissue sections by use of a technique called terminal transferase-mediated dUTP-biotin nick end-labeling (TUNEL). This technique involves the end-labeling of DNA in tissue sections with a fluorescent marker. Because the fragmented DNA in apoptotic cells has many free ends, TUNEL-labeled cells incorporate more of the fluorescent probe and glow brightly.
Mitochondria are critically involved in the regulation of apoptosis. The triggering of apoptosis is mediated by altering ratios of proapoptotic and antiapoptotic members of the B-cell lymphoma protein 2 (Bcl-2) family of proteins. Proapoptotic Bcl-2 family members promote cell death by permeabilizing the outer membrane of mitochondria and releasing numerous pro-death proteins, including cytochrome c, which is normally a crucial component of the electron transport chain, into the cytoplasm (see figure in 18–1). These pro-death proteins cannot exert their actions when contained in the intramembrane space between the inner and outer membranes of the mitochrondria due to the actions of antiapoptotic members of the Bcl-2 family. However, once in the cytoplasm, the released mitochondrial proteins promote cell death by systematically dismantling specific targets important to cell functioning. For example, cytochrome c combines with cytosolic binding partners to activate a molecular complex termed the apoptosome. The apoptosome in turn activates a family of proteases, termed caspases, which systematically cleave numerous cellular proteins.
Caspases (short for cysteine aspartate proteases) cleave and inactivate prosurvival proteins and activate proapoptotic proteins in an amplifying cascade that leads to the rapid fragmentation of cell structures (see 18–1). Under basal conditions, caspases exist as inactive proenzymes until they are activated by proteolytic cleavage. After it enters the cytoplasm, cytochrome c triggers the cleavage and activation of caspase-9. Activation of caspase-9 then triggers the cleavage and activation of several other caspases including caspases-3, -6, and -7. After they are activated, caspases cleave numerous types of proteins that trigger fragmentation of DNA, cytoskeletal proteins, and other cell structures.
Researchers are now trying to determine whether interference with apoptotic processes will improve treatment outcomes, such as decreasing the area of infarction caused by stroke. For instance, overexpression of the antiapoptosis Bcl-2 gene, or knockout of the proapoptosis Bax gene, reduces infarction in rodent brains subjected to focal ischemic insults (see Chapter 20 for further discussion of stroke). These findings indicate that inhibition of apoptotic pathways may indeed be neuroprotective. New treatments have focused on combining apoptosis inhibition with additional agents, such as antioxidants, neuronal growth factors, ion channel blockers, or thrombolytic compounds to achieve synergistic benefits.
Caspase inhibitors offer an attractive target for pharmaceutical development in part because of the relative ease in designing a drug to inhibit the active site of a protease. Some of the most effective treatments for human immunodeficiency virus (HIV) are aspartyl protease inhibitors. Currently available caspase inhibitors consist of small peptides that do not readily cross the blood–brain barrier or enter cells. Efforts are under way to develop small molecule inhibitors that might overcome such limitations. However, as stated earlier, such inhibitors must be developed with caution, given the evidence that apoptosis is important in protecting the organism from damaged and potentially cancerous cells. Under some circumstances, interference with apoptosis may worsen stroke-related damage. If neurons were to undergo necrosis instead of apoptosis, increased damage to healthy neighboring neurons might result.
One form of cell death unique to neurons is excitotoxicity—a pathologic process by which nerve cells are damaged and killed by excessive stimulation by excitatory neurotransmitters such as glutamate (Chapter 5). It has morphologic and biochemical features of both apoptosis and necrosis and involves increased mitochondrial membrane permeability as a critical event. Excitotoxicity can occur in response to a variety of neurologic insults, both acute and chronic. In acute injury events such as stroke, damaged neurons within the lesion site spill glutamate into the extracellular space, where glutamate can stimulate presynaptic glutamate receptors to enhance the release of additional glutamate. The resulting excessive excitation of nearby neurons is deleterious by setting in motion a series of biochemical cascades, mediated in part by excessive flux of Ca2+ into the cells, which lead to disruption of cellular function and eventually neuronal death. Additionally, in chronic neurodegenerative conditions, the ability of neurons to handle Ca2+ flux is compromised, further contributing to excitotoxicity.
When blood flow to brain tissue is interrupted, for example, during a stroke, conduits through which oxygen and nutrients enter cells, and potentially toxic metabolites exit, are severed. Interference with blood flow triggers many biochemical changes in the extracellular environment of the affected area of the brain. These changes lead rapidly to a large number of cellular disruptions including depletion of adenosine triphosphate (ATP) stores, loss of ion gradients, release of glutamate, Ca2+ pouring into cells through open channels, and activation of numerous second messenger systems. Many of these biochemical changes are very harmful to neurons and contribute to the neuronal death that results from prolonged ischemia. However, some of the sequelae of ischemia may have little effect on neuronal survival and some may even have neuroprotective effects, representing homeostatic responses to ischemic insult that neurons produce in self-defense. Accordingly, strategies designed to minimize infarct-related neural damage must be based on a clear understanding of how each ischemia-related process affects neuronal survival 18–1.
Under normal conditions, the brain uses oxygen and glucose to produce ATP through the processes of glycolysis and oxidative phosphorylation. Complete interruption of brain blood flow can deplete the brain’s supply of oxygen within 10 seconds. In the absence of oxygen, oxidative phosphorylation ceases, and anaerobic glycolysis becomes the only source of ATP for neurons. Anaerobic glycolysis supplies less than 10% of the ATP garnered from complete oxidation of glucose and leads to the production of lactic acid. Moreover, without incoming blood to supply glucose, neurons must rely on the glycolytic processing of preexisting stores of glucose and glycogen. During ischemia, these reserves are depleted within 2 to 3 minutes. Phosphocreatine stores also are consumed during this time. Consequently, ischemia can lead to the depletion of neuronal ATP within minutes. Because the basal metabolic activity of neurons is so great, such depletion rapidly leads to serious consequences.
One of the most important consequences of the loss of available ATP is the failure of ATP-dependent ion pumps and the subsequent dissipation of the resting membrane potential of neurons. As discussed in Chapter 2, the maintenance of the resting membrane potential is ultimately dependent on Na+-K+-ATPase, which exports Na+ and imports K+ against their concentration gradients at the cost of ATP. Although very large neurons can maintain a negative resting potential for some time after the inhibition of Na+-K+-ATPase, small and highly branched neurons of the mammalian brain are extremely vulnerable to loss of Na+-K+-ATPase activity because of their very large surface area to volume ratio. Accordingly, many CNS neurons depolarize rapidly after ATP depletion.
Loss of Na+-K+-ATPase activity has other consequences in addition to depolarization. Many ion pumps, including the Na+–Ca2+ exchanger, are dependent on the transmembrane Na+ gradient for their activity 18–2. As this gradient declines, so does the activity of these exchange systems, which leads to further disruptions of ionic equilibrium. Moreover, many ATP-dependent ion pumps, such as ATP-dependent Ca2+ pumps, are disabled when neuronal energy stores are depleted.
18–2
Ca2+ homeostasis in neurons. 1. Ca2+ is removed from the cytoplasm by Ca2+–Na+ exchangers and adenosine triphosphate (ATP)–dependent pumps. 2. Ca2+ is buffered by intracellular anions (A–). 3. Subsequently it is sequestered in organelles, such as mitochondria (3a) and endoplasmic reticulum (3b), in an ATP-dependent fashion. During ischemia, Ca2+ pumps fail because of the lack of ATP. 4. Decreases in membrane potential lead to Ca2+ entry through voltage-dependent Ca2+ channels. Release of glutamate by neighboring cells permits Ca2+ entry through NMDA and other Ca2+-conducting glutamate receptors 5. and activates Gq-coupled metabotropic glutamate receptors 6. which increase the formation of inositol triphosphate (IP3) and the release of Ca2+ from internal stores (eg, endoplasmic reticulum). ADP, adenosine diphosphate; PLC, phospholipase C; PIP2, phosphatidylinositol bisphosphate; IP3R, IP3 receptor; DAG, diacylglycerol.

As neurons depolarize they fire more action potentials and, as such firing escalates, further depolarization occurs, causing a still further rundown of the many ionic gradients required for normal neuronal function. Increased neuronal firing and increased cellular levels of free Ca2+ trigger the uncontrolled release of neurotransmitters including the powerful excitatory neurotransmitter, glutamate (Chapter 5). Released glutamate can then trigger neighboring neurons to depolarize and fire action potentials, further extending the injurious chain of events.
Neural damage caused by excessive release of glutamate is potentiated by the failure of glutamate transporters. As explained in Chapter 5, these transporters, which are expressed mainly by glial but also by neural cells, assist in terminating the actions of synaptically released glutamate by removing glutamate from the synaptic cleft. This reuptake process depends on ATP; thus, when ATP stores in the brain become depleted in response to ischemia, the functioning of these transporters is compromised. Subsequently, higher than normal levels of glutamate accumulate in synapses and cause activation of glutamate receptors for abnormally long periods of time.
The role of glutamate in excitotoxicity has spurred the investigation of drugs that block glutamate action in situations of ischemia such as stroke (Chapter 20). Early studies evaluated the utility of potent NMDA receptor antagonists, such as MK-801. However, all of these drugs can induce cognitive distortions, dissociation, and hallucinations. Indeed, phencyclidine (PCP), also known as angel dust, is abused to produce these symptoms (Chapter 16). Other well-known NMDA receptor antagonists include ketamine and dextromethorphan. Several synthetic opioids have NMDA-antagonist properties including meperidine and tramadol. Unfortunately, it has not been possible to separate the neuroprotective effects of potent NMDA antagonists from their unwanted psychotropic actions (Chapter 17). One potential intervention is memantine, a weaker NMDA antagonist, although the clinical efficacy of memantine is limited, and the mechanism for these modest clinical effects in Alzheimer disease is likely unrelated to NMDA receptor blockade at the dosages utilized clinically as discussed further below.
Under normal conditions, concentrations of cytosolic Ca2+ are under extremely tight regulation (Chapter 2) with measured increases in Ca2+ influx being crucial for numerous cellular functions (Chapter 4) but excessive Ca2+ influx causing toxicity. In a neuron at rest, Ca2+ concentrations are approximately 100 nM, whereas extracellular Ca2+ concentrations are in the millimolar range. With extracellular Ca2+ approaching concentrations that are 10,000 times greater than cytosolic concentrations, there is normally a tremendous driving force for Ca2+ to enter the neuronal cytoplasm. The great effort that a neuron expends in maintaining a low cytosolic Ca2+ concentration is compromised during ischemia (see 18–2). Ca2+ that enters the cytosol is removed by a variety of mechanisms, including the ATP-dependent Ca2+ pumps and Na+–Ca2+ exchangers previously mentioned. Both of these pumps are ultimately ATP-dependent because ATP-dependent maintenance of the Na+ gradient is required for the effective performance of the Na+–Ca2+ exchanger; indeed, as the Na+ gradient dissipates, it may even reverse and cause Ca2+ to be pumped into the cell. Depolarization also can lead to the opening of various voltage-gated Ca2+ channels, and glutamate release from neighboring cells can induce the entry of Ca2+ through NMDA and certain AMPA glutamate receptors. As a result, Ca2+ channel blockers, alone or in combination with other agents, are being studied as potential novel treatments for excitotoxicity, such as that seen during stroke (Chapter 20).
Increases in neuronal Ca2+ may be the ultimate cause of ischemia-related neuronal death (see 18–1). When Ca2+ entry is restricted in vitro, for example, through the removal of Ca2+ from the extracellular medium or through the blockage of certain Ca2+-conducting ion channels, neurons are protected against excitotoxic damage. Moreover, neurons that express subtypes of AMPA receptors that conduct Ca2+ (ie, GluA2 lacking receptors; Chapter 5) exhibit heightened vulnerability to glutamate receptor–induced toxicity. Why does a high cytosolic Ca2+ concentration induce neuronal death? This question remains under investigation, but there are several clues to the answer. One clue lies in the fact that Ca2+ functions as a signaling molecule (Chapter 4). As described in previous chapters, many neuronal enzymes, and hence many neural processes, respond to this signal. It is possible that excessive activation of these various enzymes and processes, caused by inappropriate Ca2+ levels in cells, may contribute to neuronal stress and death. Identification of the key proteins involved would help drug discovery efforts to potentially intervene therapeutically.
Among the many enzymes that have been implicated in Ca2+-mediated neuronal damage are Ca2+-activated proteases, particularly calpain, which can produce widespread cellular damage. Calpain cleaves cytoskeletal proteins that leads to severe cytoskeletal damage. Phospholipases, such as phospholipase A2 (PLA2), also can be activated by Ca2+. PLA2 targets membrane phospholipids and hydrolyzes the bond between the fatty acid portions and the polar head groups. When activated en masse, phospholipases may disrupt membrane integrity by changing the numbers and proportions of phospholipids in the neuronal membrane. Moreover, the resulting free fatty acids, which include arachidonic acid, are metabolized by cyclooxygenases and lipoxygenases to yield numerous biologically active products, such as prostaglandins (Chapter 4). The highly reactive •O2− radical is formed as part of these reactions.
Nitric oxide synthase (NOS), which is activated on binding to Ca2+–calmodulin complexes, produces gaseous NO from arginine. As explained in Chapter 4, NO is an important messenger molecule; among its other actions, it activates guanylyl cyclase, which catalyzes the formation of cGMP. NO also can inhibit many cellular enzymes, particularly those containing iron–sulfur complexes, such as mitochondrial NADH–ubiquinone oxidoreductase and NADH–succinate oxidoreductase that are crucial to metabolism. NO produces some of these effects by nitrosylating specific target proteins. Other reactions of the NO molecule—especially its reaction with oxygen to yield peroxynitrate—also may be important in neuronal demise because they may lead to the formation of free radicals.
In vitro studies have demonstrated that excitotoxicity is frequently associated with the production of large numbers of free radicals. Free radicals are molecules with an unpaired electron in an outer shell; this unpaired electron causes the molecule to be unstable and highly reactive. Free radicals can peroxidize membrane fatty acids, and in turn can induce alterations in membrane fluidity and integrity. They also can oxidize protein sulfhydryl groups, the products of which can subsequently be metabolized to yield a variety of products, including •O2–. Activation of NOS by Ca2+ increases levels of NO, which can react with oxygen or O2− to yield peroxynitrate (ONOO); ONOO decomposes with the production of •OH, a highly toxic free radical.
Regardless of the source, many experiments have shown that free radical scavengers protect against excitotoxicity in vitro. Such scavengers may effectively reduce ischemic injury only when given soon after the occurrence of an ischemic event and may be good candidates to combine with thrombolytic medications (which dissolve blood clots; Chapter 20). Only a small number of free radical scavengers have been investigated in clinical trials of stroke, and none have yet been shown to be efficacious.
Interruption of blood supply to the brain leads to both increased CO2 tension—due to decreased CO2 removal—and increased lactate accumulation caused by forced glycolysis in the absence of aerobic respiration. These two events result in acidosis. Ischemia-related acidosis can be severe; indeed, pH values in an ischemic region often approach 6.0. Acidosis affects cellular function in multiple ways, including the promotion of free radical formation; inhibition of the Na+–Ca2+ exchanger; decomposition of nicotinamide–adenine dinucleotide (NAD), which is essential for oxidative phosphorylation; and inhibition of neurotransmitter reuptake.
Despite our knowledge of these effects, the significance of acidosis to the ultimate death or survival of ischemic neurons is unclear. In fact, acidosis may have certain neuroprotective functions. Neurons exposed to high levels of glutamate in vitro undergo injury at a neutral pH; however, much of this damage is avoided when such experiments are performed at an acidic pH of 6.6. Although many plausible explanations for the protective effect of acidosis exist, one of the most likely involves the pH sensitivity of the Ca2+-conducting NMDA receptor. At acidic pH, NMDA currents are drastically reduced, which may in turn significantly reduce Ca2+ accumulation in challenged neurons.
Despite widely divergent etiologies, many neurodegenerative diseases are characterized by the inappropriate accumulation of protein aggregates. The protein aggregates can occur either intracellularly in diseases such as Parkinson disease, Huntington disease, Alzheimer disease (ie, neurofibrillary tangles), ALS, and spinocerebellar ataxia type 1 or extracellularly as in the amyloid-β–containing plaques of Alzheimer disease. Although precise mechanisms have yet to be determined, these protein aggregates have been shown to interfere with a wide variety of intracellular processes that can be deleterious to the functioning of the cell.
The proteasome endopeptidase complex has been likened to an intracellular “trash can.” When a protein is no longer necessary for cellular functioning, it is targeted for destruction by polyubiquitination, the enzymatic addition of small proteins called ubiquitin by enzymes called ubiquitin ligases 18–3. Once a protein has been tagged with four or more ubiquitins, it is transported to the proteasome, unfolded, and cleaved into short peptides, which can then be further degraded into amino acids by cytosolic peptidases.
18–3
Ubiquitin-dependent degradation of proteins by the proteasome. E1 ligases activate ubiquitin (U) (Step 1), and the activated ubiquitin is transferred to E2 ligases that recruit E3 ligases bound to specific substrate proteins (S) (Step 2). This leads to ubiquitination of the substrate on specific lysine residues (Step 3). The process is repeated as the substrate is polyubiquitinated (Step 4). The polyubiquitinated protein is then digested in the proteasome complex.

As will be seen below, several neurodegenerative disorders (eg, Huntington disease, several spinocerebellar ataxias) are characterized by the genetic expansion of a chain of glutamine residues within a particular protein. These proteins can undergo polyubiquitination, but are unable to be processed by the proteasome due to inefficient cleavage between glutamine residues. Another example for proteasome involvement in neurodegeneration comes from identification of one particular ubiquitin ligase, parkin, as a gene involved in a rare form of Parkinson disease. Loss of function in the parkin gene decreases the ability to add ubiquitin to mitochondrial outer membrane proteins. This in turn appears to disrupt mitochondrial homeostasis. Diminished proteasome activity, by either accumulation of uncleavable aggregates (as with polyglutamine diseases) or genetic loss of function of ubiquitinating enzymes (as with parkin mutations), results in a buildup of unwanted and potentially toxic proteins and organelles within the cell. Intensive research efforts are now aimed to identify the mechanism by which protein aggregates lead to neuronal death and to develop novel methods to intervene to limit such mechanisms of neurodegeneration.
The endoplasmic reticulum (ER), an intracellular system of membranes (Chapter 2), has unique mechanisms for managing protein aggregates. Unfolded or misfolded proteins are recognized and bound by ER chaperone proteins. However, when all available chaperones are completely bound by misfolded proteins, chaperone-associated signaling proteins are released and activate a process termed the “unfolded protein response.” The unfolded protein response functions to help the cell adapt to the increased load of misfolded protein. Part of this response is to halt protein translation and enhance protein degradation. In neurodegenerative diseases associated with intracellular protein aggregates, it is possible that these normal homeostatic mechanisms for handling misfolded proteins are overwhelmed, enabling the proteins to aggregate and damage the cells in other ways. There is also evidence that chronic activation of the unfolded protein response can induce apoptosis through a mitochondrial pathway dependent on proapoptotic Bcl-2 family members (see 18–1).
Autophagy refers to a cellular method for clearing items too large for proteasome degradation, such as damaged organelles or large protein aggregates. In this process the target is engulfed by an autophagosome that then fuses to a lysosome to allow for degradation of the target by lysosomal enzymes. In addition to eliminating large structures, autophagy is used by the cell to free amino acids for synthesis of critical proteins during periods of protein starvation. While this process is initially adaptive for the cell, excessive activation of autophagy can lead to a form of programmed cell death through inappropriate degradation of proteins and organelles. Abnormal autophagy has been implicated in Parkinson disease (see below) as well as in other neurodegenerative diseases. Importantly, pharmacologic inhibition of apoptotic caspases has been shown in some systems to induce autophagy, indicating that redundant pathways exist to dispose of damaged cells.
Several nonspecific strategies to treat abnormal, pathogenic protein aggregation are currently in development. Most approaches involve the use of drugs that prevent protein aggregation in cultured cells or in mouse models of neurodegeneration. Some medications in this class include cystamine, geldanamycin, and minocycline, which are now in clinical trials. Cystamine forms disulfide bonds with and inhibits several enzymes, including certain caspases. Geldanamycin activates heat shock protein-90, an important chaperone, which, by binding to toxic proteins, may protect cells from proapoptotic signals. Although the molecular mechanism of action of minocycline is unknown, the drug has been shown to inhibit microglial activation in vitro and in vivo.
Another potential therapy currently under study in Huntington disease is rapamycin that can induce autophagy and potentially speed the clearance of toxic aggregates. Rapamycin acts by inhibiting mTor (mammalian target of rapamycin), a protein downstream of several neurotrophic factor signaling pathways, including AKT (see figure in 18–1; see also Chapters 4 and 8), which exerts powerful control over protein synthesis and autophagy. Because of their central role in apoptosis, medications that stabilize mitochondrial function, such as coenzyme Q10 and creatine, are of great interest. Both compounds are antioxidants.
Recent work has implicated the generation of toxic peptides from protein aggregates in the pathogenesis of neurodegeneration. For example, mice carrying mutant huntingtin (the cause of Huntington disease; see below) exhibit increased cell death. When the caspase recognition sites in the mutant protein are altered to prevent their cleavage by caspases, neurons become resistant to cell death in these mouse models. These findings suggest that release of toxic peptides by proteolytic cleavage of protein aggregates may be important for the progression of certain neurodegenerative diseases. The findings further substantiate the potential utility of caspase inhibitors in the treatment of these illnesses.
Alzheimer disease has become a major public health problem as human life span has been extended by modern medicine. It is the most common cause of dementia and is characterized by a gradual decline in memory and other cognitive functions over a period of years. The disease processes in the brain begin about 15 years prior to the appearance of symptoms, which are generally noticed later in life. Among individuals older than 65 years of age, 6% to 8% have Alzheimer disease; among persons aged 85 and older, the prevalence of Alzheimer disease is approximately 50%. Rare monogenic forms of the disease can begin in midlife.
Among the earliest symptoms of Alzheimer disease are memory impairment and problems with executive function (problem solving). As Alzheimer disease advances, the ability to learn new information is increasingly compromised. Access to distant memories, which is relatively intact in the initial stages of the disease, begins to diminish. As cognitive impairment progresses, patients may become lost while walking or driving, and become increasingly unable to perform tasks such as preparing meals, taking regular medications, or managing finances. As cognition declines, patients may experience depression and irritability and later delusions and hallucinations. Patients also may become aggressive, even toward their caretakers. The end stage of Alzheimer disease is generally characterized by a complete loss of independence. The disease exacts an enormous toll from not only affected individuals but also the friends and family members who care for them and society at large.
As the number and percentage of elderly persons increase steadily, the prevalence of Alzheimer disease almost certainly will rise. It is estimated that by the year 2040, there will be 11 million people in the United States and 80 million people worldwide with Alzheimer disease. Thus, there is an enormous need to develop effective medications to slow or halt the disease process.
Even cursory inspection reveals that the brain of a patient with mid- to late-stage Alzheimer disease is noticeably different from that of a normal person of the same age. The diseased brain appears shrunken compared with the normal brain, and has wider sulci, larger ventricles, and less overall mass. However, the pathologic hallmarks of Alzheimer disease and clues as to its etiology can be observed only at the microscopic level. In addition to loss of neurons and synapses, these hallmarks include the presence of various abnormal protein deposits. Senile plaques are dense, extracellular deposits that are composed primarily of the 38- to 43-amino-acid amyloid-β peptide (Aβ), the biochemistry of which is discussed later in this chapter. Two types of senile plaques—diffuse plaques and neuritic plaques—have been identified. Diffuse plaques are extracellular deposits of Aβ in which the Aβ peptide is aggregated but is not present in a β-sheet conformation. Neuritic plaques also consist of extracellular masses of Aβ, but are distinguished from diffuse plaques by the presence of Aβ in a β-sheet conformation as well as the presence of dystrophic dendrites and activated astrocytes and microglia. Intracellular accumulations of abnormally phosphorylated helical filaments in the form of neurofibrillary tangles and neuropil threads also are hallmarks of Alzheimer disease. The major constituent of the tangles is the microtubule-associated protein tau, a protein present in normal brain tissue that is both abnormally phosphorylated and aggregated in Alzheimer disease.
For reasons that currently are not entirely clear, tangle formation and neuron loss tend to develop preferentially in the hippocampus and medial temporal neocortex, and plaques form first in the frontal, parietal, and other association cortices. The distribution of plaques may be related to the relative amount of neuronal and synaptic activity that occurs over a lifetime. This is supported by data showing that Aβ levels are regulated by synaptic activity and that the highest levels of Aβ and subsequent amyloid plaque development occur in brain regions with the highest levels of neuronal activity such as in a cortical circuit known as the default mode network. Cholinergic nuclei such as the nucleus basalis (Chapter 6) are highly impaired while primary sensory cortices and subcortical brain regions tend to be spared. This pattern correlates with early onset memory impairment (declarative memory is dependent on the medial temporal lobe) and progressive cognitive decline (Chapter 14).
The significance of the defining pathologic features of Alzheimer disease has been the topic of intense research. Among the most important questions that investigators have attempted to answer are those related to causality. Are plaques and tangles toxic to neurons? Or are they coping mechanisms that the cell uses to detoxify other, more toxic forms of Aβ and tau? While answers to these questions are still unclear, there is increasing evidence that small, soluble oligomers of Aβ (ranging from dimers to dodecamers and larger species) are particularly pathogenic and may be a major instigator of Alzheimer disease, whereas tau aggregation and its spread to different regions of the brain may be critical in the progression of cognitive loss.
Two primary risk factors for Alzheimer disease have long been recognized: advanced age and genetics. Common forms of Alzheimer disease begin later in life and appear to be genetically complex. There are, however, inherited forms in which symptoms appear in middle age and follow autosomal dominant inheritance patterns. More than 100 different mutations in three known genes (amyloid precursor protein [APP], presenilin-1, and presenilin-2) can each cause early onset, familial Alzheimer disease, with some differences in age of onset and rate of progression.
In late-onset forms, the disease does not occur in a Mendelian inheritance pattern. Nonetheless, family studies have revealed that between 25% and 50% of relatives of patients with Alzheimer disease eventually are afflicted with the disease themselves, compared with approximately 10% among control groups. The apolipoprotein E (APOE) gene is the most important known genetic factor that modifies the risk for Alzheimer disease and the age at which the disease emerges. The APOE4 allele increases risk, whereas the APOE2 allele decreases risk, relative to the APOE3 allele. Numerous other genes identified in genome-wide investigations appear to modify the risk for Alzheimer disease, although none yet have been shown to exert as large an effect as APOE. Environmental factors such as sleep and exercise are probably also important in determining whether or not an individual will develop the disease.
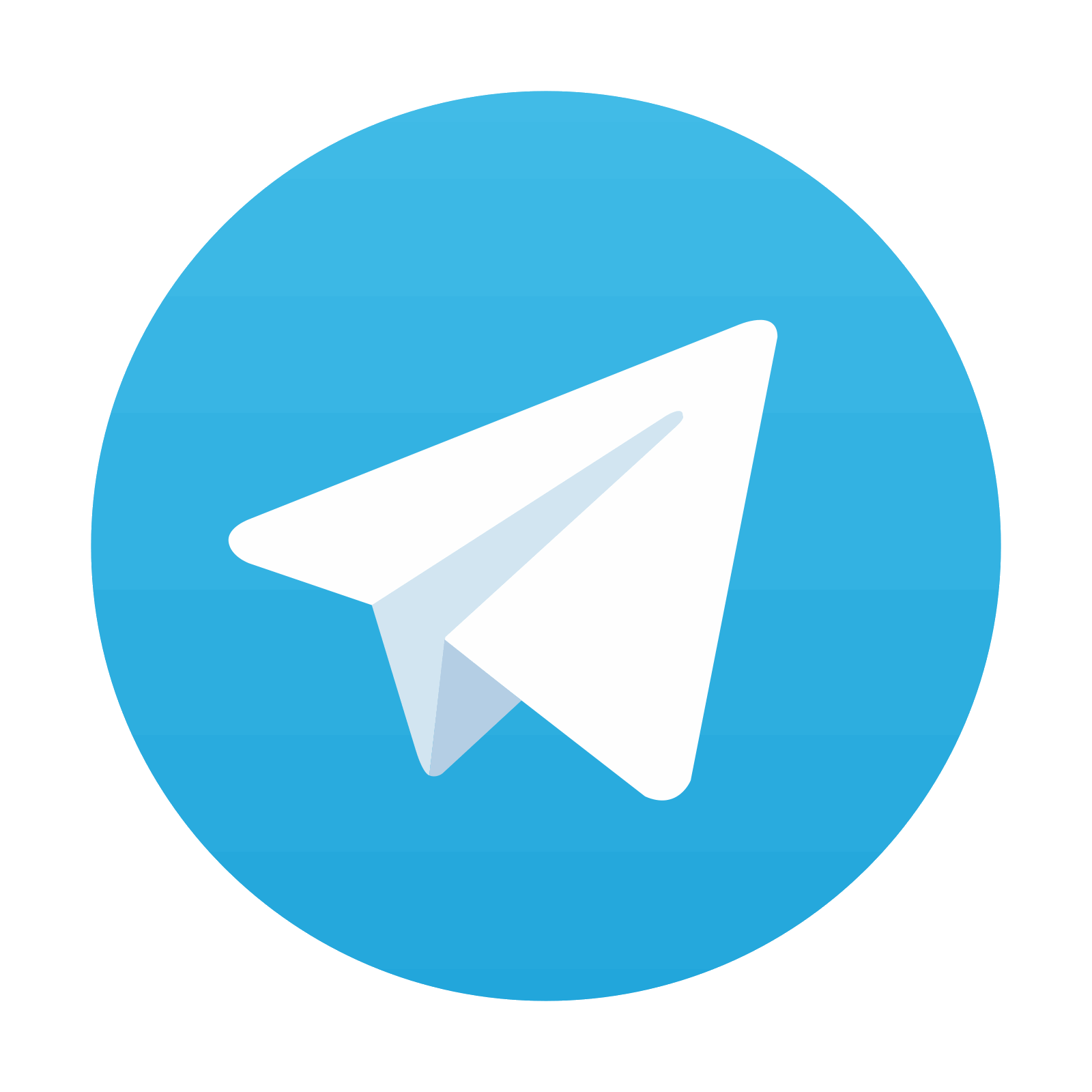
Stay updated, free articles. Join our Telegram channel
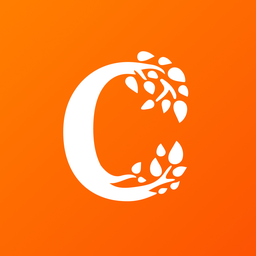
Full access? Get Clinical Tree
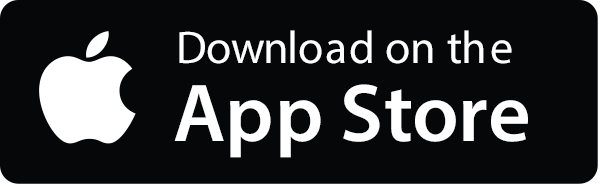
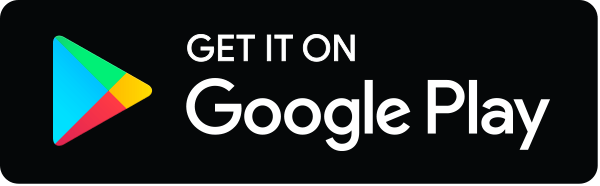