Signal transduction refers to the processes by which signals between cells carried by neurotransmitters, hormones, trophic factors, and cytokines are converted into biochemical signals within cells.
Most neurotransmitter receptors can be divided into two classes by their signal transduction mechanism—one class involving activation of an ion channel that is intrinsic to the receptor and the other involving activation of G proteins.
Signal transduction can alter neuronal function on vastly different time scales ranging from very rapid (millisecond) changes in membrane potential produced by ligand-gated channels to changes over seconds produced by intracellular second messengers and protein kinases.
Many critical drugs that act on the nervous system are agonists or antagonists at G protein–coupled receptors.
Although second messengers such as cyclic nucleotides and Ca2+ may directly gate ion channels, their major role in intracellular signaling systems is to regulate protein serine–threonine kinases that phosphorylate other proteins.
The brain contains numerous other types of protein serine–threonine kinases that also play important roles in the regulation of cell function.
Neurotrophins, such as nerve growth factor and brain-derived neurotrophic factor, interact with a family of receptors termed receptor tyrosine kinases (Trks).
Certain cytokines act on receptors that activate Janus kinases, which in turn activate a family of transcription factors called signal transducers and activators of transcription (STATs).
Protein phosphatases, also categorized into serine–threonine or tyrosine subfamilies, reverse the actions of protein kinases and serve critical functions in cell regulation.
Many intracellular signaling pathways ultimately regulate gene expression.
Transcription is stimulated when an activator protein displaces nucleosomes, the major component of chromatin, permitting a complex of proteins, called general transcription factors, to bind DNA at a core promoter and recruit RNA polymerase.
DNA-binding sites for regulatory proteins are called regulatory elements, and the proteins that bind them are called transcription factors.
Each gene has a unique pattern of cellular expression and response to physiologic signals based on the combinatorial interaction of regulatory elements found within its regulatory regions.
Eukaryotic cells increase the diversity of proteins that can be produced from a single gene by alternatively splicing the exons within the primary transcript.
Mature (spliced) messenger RNAs are transported from the nucleus into the cytoplasm, where they are translated to proteins on organelles called ribosomes.
During and after translation, proteins are processed by cleavage into smaller proteins and by a variety of covalent modifications such as glycosylation.
Numerous families of transcription factors, with diverse regulatory and functional properties, control gene expression in the CNS under normal and pathological conditions.
Signal transduction refers to the processes by which intercellular signals such as neurotransmitters, neurotrophic factors, circulating hormones, and cytokines produce intracellular biochemical alterations that in turn modify neuronal functioning including the regulation of gene expression. Intercellular signals and their receptors, generally located in the neuronal plasma membrane, represent only a small amount of all information processing in the brain. An understanding of the complex biochemical mechanisms that operate inside neurons and ultimately mediate the actions of all intercellular signals is required to appreciate not only the ways in which the brain responds to drugs and other stimuli but also the ways in which it continuously adapts to a host of environmental changes.
Four general patterns of signal transduction occur in the brain 4–1. One pattern 4–1A, discussed briefly in Chapter 3, involves the binding of neurotransmitter to a multimeric plasma membrane receptor complex that contains a ligand-gated ion channel. Protein–protein interactions tether such ion channels, or receptor ionophores, at proper subcellular locations and often to other signaling proteins. This mechanism of signal transduction is used primarily by the amino acid neurotransmitters at their ionotropic receptors (Chapter 5) as well as by acetylcholine at nicotinic receptors (Chapter 6), serotonin at 5HT3 receptors (Chapter 6), adenosine triphosphate (ATP) at P2X purinergic receptors (Chapter 8), and heat and many other environmental signals at transient receptor potential (TRP) channels (Chapters 2 and 11). The structural features of ligand-gated ion channels are discussed in detail in the chapters devoted to these transmitters.
4–1
General patterns of signal transduction in the brain. A. Neurotransmitter activation of a receptor that contains an ion channel. B. Neurotransmitter activation of a G protein–coupled receptor. After it is activated, the G protein can directly regulate an ion channel (left), or trigger the regulation of second messenger–dependent protein kinases and protein phosphatases, which can in turn regulate other ion channels and many neuronal processes (right). C. Neurotrophic factor activation of a receptor that contains protein tyrosine kinase activity or that activates such a kinase indirectly. D. Steroid hormone activation of a cytoplasmic receptor. After the receptor is bound by hormone, it translocates to the nucleus and regulates gene expression.

A second pattern, also briefly described in Chapter 3, is characterized by the binding of neurotransmitter to a plasma membrane receptor that couples with a guanine nucleotide–binding protein or G protein 4–1B. Most neurotransmitters bind to this superfamily of G protein–coupled receptors, as do several cytokines. All such receptors have a seven-transmembrane domain structure, whose N terminus faces the extracellular space and whose C terminus faces the cytoplasm; other structural features of these receptors are discussed in detail in Chapter 6, wherein the β-adrenergic receptor is presented as a prototype. The binding of ligands to these receptors initiates a range of biologic effects on target neurons, which include direct G protein regulation of certain ion channels (see 4–1B, left) and the triggering of complex cascades of intracellular messengers. Activation of intracellular messenger pathways leads to the generation of second messengers and the regulation of protein phosphorylation, and ultimately to diverse physiologic responses to extracellular stimuli 4–2, including regulation of ion channels (see 4–1B, right). As will become evident, protein phosphorylation is the major molecular currency of intracellular signal transduction pathways. Protein phosphorylation describes a process by which protein kinases add phosphate groups to specific target proteins, and protein phosphatases (PPPs) remove these phosphate groups. Phosphate groups, because of their large size and negative charge, alter the conformation and overall charge of a protein and hence its function.
4–2
Signal transduction pathways in neurons. Extracellular signals, or first messengers, produce biologic responses in target neurons through a series of intracellular signals. Many activate second messenger pathways resulting in the phosphorylation of diverse types of proteins that can be considered third messengers, which mediate the biologic responses of the first messengers. First messengers also produce biologic responses through the activation of protein tyrosine kinases, some of which are physically linked to plasma membrane receptors (R), whereas others are cytoplasmic.

A third pattern of signal transduction involves the direct activation of a class of protein kinases, called protein tyrosine kinases, which phosphorylate proteins on tyrosine residues 4–1C. This type of signaling is used by most types of neurotrophic factors and cytokines. In some cases the neurotrophic factor receptor and the protein kinase reside in a single protein; in other cases the receptor must recruit a cytoplasmic kinase to effect its signaling (see 4–2). Activation of the protein tyrosine kinase triggers cascades of further protein phosphorylation that ultimately lead to the many effects of neurotrophic factors on brain function, including the regulation of ion channels.
A fourth pattern characterizes transduction by all known steroid hormones and certain other lipophilic extracellular signals that cross the plasma membrane and activate receptors in the neuronal cytoplasm 4–1D. On binding their ligand, these cytoplasmic receptors translocate to the nucleus, where they bind DNA and function as transcription factors. Thus, these receptors can be considered ligand-activated transcription factors.
Much of the specificity and rapidity of cell signaling comes from the spatial apposition of signaling proteins in microdomains of the cell’s plasma membrane termed lipid rafts. These specializations, which are rich in cholesterol and sphingolipids, organize sets of signaling proteins into discrete compartments, which greatly facilitates complex signaling cascades.
G proteins perform a central function in the process of transmembrane signaling in the nervous system. These proteins were named because of their ability to bind the guanine nucleotides, guanosine triphosphate (GTP), and guanosine diphosphate (GDP). G proteins couple receptors to specific intracellular effector systems (see 4–1). Three major types of G protein are involved in the transduction of signals produced by neurotransmitter binding: Gs, Gi/o, and Gq 4–1. Other subtypes serve more specialized functions in discrete cell types. Each type of G protein is a heterotrimer composed of single α, β, and γ subunits. Individual αs, αi, αq, etc, subunits are primarily responsible for the unique functions of the G proteins that contain them 4–1. Additionally, several types of β and γ subunits complex with one another and differentially associate with subtypes of α subunits to confer still greater degrees of specificity on intracellular signaling proteins. The functional cycle of G proteins is shown schematically in 4–3. All eukaryotic cells also contain a distinct class of monomeric guanine nucleotide–binding proteins that subserve numerous critical roles in the regulation of cell function; these are termed small-molecular-weight G proteins and are described in 4–1.
Class | Molecular Mass (kDa) | Toxin-Mediated ADP-Ribosylation | Effector Protein |
---|---|---|---|
Gαs family (includes Gαolf) | 45–52 | Cholera | Adenylyl cyclase (activation) |
Gαi family (includes Gαgust, Gαt, and Gαz) | 39–41 | Pertussis | Adenylyl cyclase (inhibition) |
Others | |||
Gαq family (includes Gα11, 14–16) | 41–43 | None | Phospholipase C (activation) |
Others | |||
Gα12 family | 44 | None | Unknown |
4–3
G protein function. A. Under basal conditions, G proteins exist in cell membranes as heterotrimers composed of single α, β, and γ subunits. The α subunits are bound to GDP, and the G protein heterotrimer is anchored to the plasma membrane by an isoprenyl (acyl) group attached to C-terminal cysteine residues of the γ subunit. B. After the receptor (R) is activated by its ligand (eg, neurotransmitter), it physically associates with the α subunit, causing the latter to release GDP. Subsequently, GTP (present in the cell at higher concentrations than GDP) binds to the α subunit. C. GTP binding causes the dissociation of the α subunit from its βγ subunits and from the receptor. Free α subunits, bound to GTP, are functionally active and directly regulate effector proteins, such as adenylyl cyclase and phospholipase C. Free βγ subunits are also biologically active and directly regulate some of the same effector proteins as well as ion channels. D. GTPase activity intrinsic to the α subunit degrades GTP to form GDP, and in turn causes the reassociation of the α and βγ subunits. This reassociation, in conjunction with the dissociation of the ligand from the receptor, restores the basal state.

4–1 Small G Proteins
A large superfamily of small G proteins, so named because of their low molecular mass (20–25 kDa), serve critical functions in the cell. Like the α subunits of heterotrimeric G proteins, small G proteins bind guanine nucleotides, possess intrinsic GTPase activity, and cycle through GDP- and GTP-bound forms 4–3. All classes of G protein undergo a change in their affinity for target molecules as they shift from GDP- to GTP-bound forms, because such a shift causes them to undergo a large conformational change. This enables small G proteins to function as molecular switches that control several cellular processes (see table).
Among the best characterized small G proteins are those that comprise the Ras family, a series of related proteins of approximately 21 kDa. Their activity is highly regulated by a variety of associated proteins (see figure). Guanine nucleotide exchange factors (GEFs) increase the activity of Ras proteins by stimulating the release of GDP from their inactive forms and facilitating their binding to GTP. In contrast, GTPase-activating proteins (GAPs) bind to Ras proteins, thereby stimulating their intrinsic GTPase activity and reducing their functional activity. A mutation in one type of GAP results in neurofibromatosis, a disease characterized by the uncontrolled growth of glial cells that express myelin. Conversely, GTPase inhibitory proteins (GIPs) bind to Ras proteins and inhibit their GTPase activity.
Ras proteins and heterotrimeric G proteins interact with their related proteins in strikingly analogous ways. For example, G protein–coupled receptors provide essentially the same function for heterotrimeric G proteins that GEFs perform for Ras proteins. Likewise, GTPase-activating and GTPase-inhibiting proteins modify Ras proteins in much the same way that RGS proteins and βγ subunits regulate heterotrimeric G proteins. However, there are important differences as well. Because Ras proteins are characterized by far less intrinsic GTPase activity than heterotrimeric G protein α subunits, GAPs exert a more profound effect on the functioning of the Ras system and are essentially responsible for turning it on and off.
Numerous types of cell signals, including most neurotrophic factors, converge on Ras and related proteins to regulate MAP-kinase pathways, which in turn produce diverse effects on cell function 4–14, 4–15.
Rho proteins, comprising Rho, Rac, and Cdc42, regulate dendritic growth and many other cell functions. Rab proteins, which are involved in membrane vesicle trafficking, represent another small G protein family. Rab subtypes, particularly Rab3, have been implicated in the regulation of exocytosis and neurotransmitter release at nerve terminals (Chapter 3). Arf is a small G protein involved in functioning of the Golgi apparatus. It appears to control the binding of a protein coat to budding vesicles, including synaptic vesicles that mediate neurotransmitter release at the synapse.
Class | Cellular Function |
---|---|
Ras | Signal transduction (control of growth factor and MAP-kinase pathways) |
Rho, Rac, Cdc42 | Assembly of cytoskeletal structures (eg, actin microfilaments), regulation of gene expression, others |
Rab | Vesicle trafficking and exocytosis in synaptic vesicles |
Rheb | Signal transduction in mTor pathways |
Arf | Assembly and function of Golgi complex; ADP-ribosylation of Gαs |
EF-2 | Regulation of protein synthesis at ribosomes |
Ran | Nuclear-cytoplasmic trafficking of RNA and protein |
Several bacterial toxins produce their pathogenic effects by regulating the activity of specific G proteins through a process termed ADP-ribosylation (see 4–1). This process involves the addition of an ADP-ribose group from nicotinamide adenine dinucleotide (NAD) to an amino acid residue in the G protein. Cholera toxin ADP-ribosylates and irreversibly activates Gαs by inhibiting its GTPase activity, whereas pertussis toxin ADP-ribosylates and inactivates Gαi and Gαo by stabilizing their association with βγ subunits. Diphtheria toxin ADP-ribosylates and inactivates a small G protein, known as eukaryotic elongation factor 2, which regulates ribosomal function (see 4–1). G proteins also are the targets of certain insect venoms. The best characterized is mastoparan, a 10 amino acid–long peptide contained in wasp venom. The peptide stimulates the GTPase activity of Gαi subunits, thereby shortening the duration of the GTP-bound activated subunits.
G protein function can be regulated by several modulatory proteins. A neurotransmitter receptor may be seen as one type of modulatory protein, specifically a guanine nucleotide exchange factor (GEF), because it triggers the release of GDP from the α subunit. Regulators of G protein signaling (RGS proteins) are GTPase-activating proteins (GAPs) that activate the GTPase activity intrinsic to the α subunits of G proteins; thus, these proteins inhibit G protein function by shortening the duration of the signals from both the activated GTP-α subunit and the free βγ subunits. All G protein α subunits, except for Gαs, are known to be regulated in this manner. More than 20 subtypes of RGS proteins, each with a characteristic expression pattern in the brain, have been identified. Some RGS proteins also exert scaffolding functions by virtue of binding other signaling proteins that together form signaling complexes for neurotransmitter receptors. Phosducin is another modulatory protein that binds to βγ subunits and thereby competes with these subunits for binding to α subunits and possibly to effector proteins.
Many types of neurotransmitter receptors regulate ion channels by means of G proteins. The process by which this regulation occurs is best established for neurotransmitter receptors that couple to Gαi or Gαo. Via G protein coupling, these receptors activate inwardly rectifying K+ channels (GIRKs) or inhibit voltage-gated Ca2+ channels, depending on the cell type involved. This regulation occurs primarily through the βγ subunits of G proteins, which directly open, or gate, GIRKs and limit the opening of Ca2+ channels in response to membrane depolarization.
G proteins also transduce the activation of neurotransmitter receptors by neurotransmitter binding to altered intracellular levels of second messengers in target neurons. Prominent second messengers in the brain include cyclic adenosine monophosphate (cAMP), cyclic guanosine monophosphate (cGMP), Ca2+, nitric oxide (NO), and the major metabolites of both phosphatidylinositol—inositol triphosphate (IP3) and diacylglycerol (DAG)—and arachidonic acid, such as prostaglandins. Altered levels of second messengers mediate the effects of receptor activation on many types of ion channels and on numerous physiologic responses.
cAMP and cGMP are classified as cyclic nucleotides because they are synthesized from ATP and GTP, respectively, through the formation of a cyclic phosphodiester ring 4–4. Neurotransmitter receptors coupled via Gs stimulate cAMP levels via activation of adenylyl cyclase, the enzyme responsible for the synthesis of cAMP 4–5. In contrast, receptors coupled via Gi inhibit adenylyl cyclase. Nine forms of adenylyl cyclase (types I–IX) have been identified, each of which exhibits a distinctive pattern of expression in brain and peripheral tissues 4–6. All but type IX are activated by the natural plant molecule, forskolin.
4–5
Cyclic AMP (cAMP) and cyclic GMP (cGMP) second messenger systems. Most extracellular messengers influence these systems through interactions with neurotransmitter receptors (R); others, including many drugs such as phosphodiesterase inhibitors that inhibit the breakdown of cAMP and cGMP, influence these second messenger systems directly. G proteins (Gs or Gi/o) are coupling factors that mediate the ability of neurotransmitter receptors to activate or inhibit adenylyl cyclase, the enzyme that catalyzes the synthesis of cAMP. Neurotransmitters typically increase cGMP levels by elevating cellular Ca2+ levels (see 4–7). Increased Ca2+ levels trigger the activation of nitric oxide synthase (NOS), which converts arginine to nitric oxide (NO). NO subsequently activates guanylyl cyclase, which catalyzes the synthesis of cGMP. After they are synthesized, the second messengers activate cAMP-dependent protein kinase and cGMP-dependent protein kinase, respectively. PKA and PKG phosphorylate an array of substrate proteins (or third messengers), whose altered physiologic activity provokes biologic responses to extracellular messengers, either directly or indirectly (eg, through intervening fourth, fifth, or sixth messengers). Alternatively, cAMP and cGMP directly gate certain types of ion channels, and cGMP can directly activate phosphodiesterases, for example, in the retina.

4–6
Regulation of adenylyl cyclase (AC) activity. Although most forms of the enzyme are activated by Gαs and forskolin, they vary in their regulation by Ca2+ and G protein βγ subunits. A. Because adenylyl cyclase types I, III, and VIII are stimulated by Ca2+/calmodulin, these enzymes typically are activated by an increase in cellular Ca2+ levels. Gαs interacts synergistically with Ca2+/calmodulin, and, in the presence of activated Gαs, type I adenylyl cyclase is inhibited by βγ subunits. Gαi mediates neurotransmitter inhibition of these adenylyl cyclases. B. Adenylyl cyclase types II and IV are not sensitive to Ca2+/calmodulin and, in the presence of activated Gαs, are stimulated synergistically by βγ complexes. The source of the βγ complexes could be Gαs itself or any of several other types of G proteins (eg, Gi, Go, Gq), depicted as Gx in the figure. C. Types V and VI are inhibited in response to phosphorylation by cAMP-dependent protein kinase (PKA) or protein kinase C (PKC). They are also inhibited by free Ca2+ and by Gαi, but are not influenced by βγ subunits.

Neurotransmitters regulate cGMP levels by means of two mechanisms. A very small number of plasma membrane receptors, such as atrial natriuretic peptide receptors, contain guanylyl cyclase that is activated when a ligand binds to the receptor. In most cases, however, guanylyl cyclase is a cytosolic enzyme that is activated by NO (see 4–5). NO, in turn, is generated via nitric oxide synthase (NOS), which is activated by Ca2+ in conjunction with the Ca2+-binding protein calmodulin. Accordingly, neurotransmitters that increase intracellular Ca2+ levels typically increase cGMP levels by generating NO. Organic nitrates that are used as vasodilators in the treatment of ischemic heart disease work by generating free NO in vascular smooth muscle, which increases cellular cGMP levels and in turn leads to muscle relaxation. NO also regulates cell function through the S-nitrosylation of other proteins (Chapter 8). Because NO is a soluble gas, it can diffuse out of the neuron in which it is synthesized and stimulate guanylyl cyclase in neighboring cells, thereby increasing cGMP levels. Thus, NO functions as both an intercellular and an intracellular messenger in the brain. The potential utility of NOS inhibitors in the treatment of stroke is described in Chapter 20. Linaclotide is a small peptide agonist of guanylyl cyclase 2C, which is approved for the treatment of irritable bowel syndrome.
cAMP and cGMP are enzymatically degraded by phosphodiesterases (PDEs), which are expressed in numerous forms in brain and other tissues. As outlined in 4–2, these enzymes differ in their relative selectivity for cAMP and cGMP and in their regulation by other cellular signals, such as by the cyclic nucleotides themselves or by Ca2+/calmodulin. At high concentrations, caffeine and related methylxanthines inhibit PDE activity, which may contribute to some of the pharmacologic effects of these drugs, particularly at higher doses.
Family | Principal Regulatory Characteristics | Preferred Substrate | Selective Inhibitors1 |
---|---|---|---|
1 | Ca2+/calmodulin-stimulated | cAMP and cGMP | 8-Ethoxymethyl-3-isobutyl-1-methylxanthine |
2 | cGMP-stimulated | cAMP and cGMP | EHNA, BAY 60-7550 |
3 | cGMP-inhibited; phosphorylation by PKA and AKT | cAMP and cGMP | Milrinone, enoximone, amrinone2 |
4 | Phosphorylation by PKA | cAMP | Rolipram, ibudilast, RO20-1724 (antidepressant-like activity in animals) |
5 | cGMP binding (likely stimulatory) | cGMP | Sildenafil, vardenafil, tadalafil (for erectile dysfunction) |
6 | Activated by Gαt (transducin) | cGMP | — |
7 | cAMP | BRL 50481 (anti-inflammatory) | |
8 | cAMP | ||
9 | cGMP | BAY 73-6691 | |
10 | cAMP-stimulated; 10A isoform highly enriched in striatum | cAMP and cGMP | Papaverine, PF-2545920 (antipsychotic-like activity in animals) |
11 | cAMP and cGMP |
Nonselective PDE inhibitors, which affect many forms of the enzyme, are associated with pervasive side effects, but progress has been made in the development of selective PDE inhibitors. Sildenafil (Viagra) and related drugs, specific inhibitors of PDE5, are prescribed for the treatment of erectile dysfunction. PDE5 is concentrated in vascular smooth muscle and especially, but not exclusively, in the vasculature of the penis. Considerable effort also has been made toward developing PDE inhibitors that are selective for isoforms of the enzyme expressed predominantly in the brain. Rolipram, for instance, inhibits all isoforms of PDE4; this drug initially showed promise as an antidepressant, but its clinical utility was limited by side effects such as nausea. However, because PDE4 comprises many subtypes (see 4–2), an inhibitor of one subtype may lead to the development of an effective antidepressant without rolipram’s side effects. Another PDE4 inhibitor, ibudilast, which also acts on other PDE subtypes, is marketed as an anti-inflammatory agent in Japan. It is being considered for several CNS indications. There is interest as well in generating inhibitors of PDE10A, which is highly enriched in striatum. Such inhibitors are being evaluated for the treatment of schizophrenia (Chapter 17).
The regulation of intracellular Ca2+ levels by neurotransmitter receptors occurs via two major mechanisms 4–7. Neurotransmitter receptor activation can alter the flux of extracellular Ca2+ into neurons in several ways: (1) Ca2+ passes directly through certain subtypes of ligand-gated channels, such as activated N-methyl-d-aspartate (NMDA) glutamate receptors and nicotinic cholinergic receptors (Chapters 5 and 6); (2) specific voltage-gated Ca2+ channels are inhibited by Gi, as previously outlined; (3) the depolarization of a neuron, which causes the activation of voltage-gated Ca2+ channels, leads to large increases in intracellular Ca2+ levels (Chapter 2); or (4) the activation of other second messenger systems may alter Ca2+ channel properties; for example, cAMP and neurotransmitters that act through cAMP can modulate voltage-gated Ca2+ channels by regulating their phosphorylation.
4–7
Ca2+ and phosphatidylinositol second messenger systems. Most extracellular messengers act on these systems, as they do on the cAMP and cGMP systems, through interactions with neurotransmitter receptors (R). However, some drugs, such as Ca2+ channel blockers and lithium, can influence these systems directly. G proteins (typically Gq) are coupling factors that mediate the ability of neurotransmitter receptors to regulate phospholipase C (PLC), which metabolizes phosphatidylinositol (PI) to form inositol triphosphate (IP3) and diacylglycerol (DAG). IP3 acts to increase intracellular levels of free Ca2+ by releasing Ca2+ from internal stores. Increased levels of intracellular Ca2+ also result from the flux of Ca2+ across the plasma membrane stimulated by nerve impulses and certain neurotransmitters. The brain contains two major classes of Ca2+-dependent protein kinases. One is activated by Ca2+ in conjunction with the Ca2+-binding protein calmodulin (CaM-kinase), and the other, protein kinase C (PKC), is activated by Ca2+ in conjunction with DAG and various phospholipids. Many of these kinases have broad substrate specificities (as indicated by the multiple arrows in the figure). Phosphorylation of substrate proteins, or third messengers, by these various Ca2+-dependent protein kinases, alters their physiologic activity and either directly or indirectly triggers biologic responses to extracellular messengers.

The second major mechanism by which neurotransmitters can increase intracellular levels of free Ca2+ is through regulation of the phosphatidylinositol system and subsequent actions on intracellular Ca2+ stores (see 4–7). Such regulation is possible because many types of neurotransmitter receptors are coupled through G proteins to an enzyme termed phospholipase C (PLC). Receptor activation of PLC is mediated most often by subtypes of Gq, whose βγ subunits are primarily responsible for this regulation.
PLC catalyzes the breakdown of phosphatidylinositol, which results in the generation of two lipid molecules that function as second messengers in the brain: IP3 and DAG (4–8 and 4–9). The most important forms of PLC in the brain are designated β and γ 4–10. The β form is predominantly responsible for mediating the effects of neurotransmitters that are coupled to Gq, and the γ form is predominantly responsible for mediating the effects of neurotrophic factors on this enzyme.
4–9
The phosphatidylinositol cycle: actions of lithium. Many neurotransmitter receptors are linked by Gq (or another G protein) to phospholipase Cβ, which hydrolyzes phosphatidylinositol-4,5-bisphosphate (PIP2) to generate two second messengers: diacylglycerol and inositol-1,4,5-triphosphate (IP3). IP3 acts to release Ca2+ from intracellular stores and subsequently is metabolized to forms that may not participate in neural signal transduction, including inositol-1,3,4,5-tetraphosphate (Ins-1,3,4,5-P4). These forms are eventually metabolized to produce three inositol monophosphates, which differ only in terms of the carbon atom to which the phosphate group is linked. Synthesis of inositol from glucose-6-phosphate also must pass through an inositol monophosphate intermediate. All inositol monophosphates are metabolized by inositol monophosphate phosphatase, an enzyme that is inhibited by therapeutic concentrations of lithium (Li+). Thus, in the presence of lithium, these monophosphates cannot be dephosphorylated to yield free inositol, which is required to regenerate phosphatidylinositol-4,5-bisphosphate. Lithium also inhibits inositol polyphosphate-1-phosphatase, an enzyme required for other metabolic steps in the recycling pathway.

4–10
Structure of phospholipase C (PLC). The two major forms of PLC in the brain are PLCβ and PLCγ. PLCβ mediates the ability of G protein–coupled receptors (linked by Gq) to stimulate the phosphatidylinositol pathway (see 4–7). PLCγ mediates the ability of neurotrophic factors to stimulate the pathway (see 4–14). Both forms contain a pleckstrin homology domain (PH) on the N terminus that anchors the enzyme to the plasma membrane, a catalytic domain (Cat) composed of two homologous units, and a C2 domain that binds to and is activated by Ca2+. PLCβ contains a larger C-terminal domain, which enables it to couple to G proteins, whereas PLCγ contains two additional PH domains and two SH2 domains, which enable it to couple to neurotrophin receptors.

PLC action on phosphatidylinositol results in the generation of free inositol-1-phosphate that, through a series of lipid phosphorylation events, is converted into active IP3 or inositol-1,4,5-triphosphate 4–9. IP3 is subsequently recycled by means of successive dephosphorylation into inositol, which cells use to replenish phosphatidylinositol in their membranes. Lithium, which remains an important drug in the treatment of mania and other mood disorders, inhibits several of these inositol phosphatases, although it is not known whether this action is related to the drug’s clinical effects (Chapter 17). IP3 acts on an IP3 receptor, which functions to release Ca2+ from intracellular organelles such as the endoplasmic reticulum. Thapsigargin inhibits this effect. Some organelles also contain a related ryanodine receptor, which triggers the release of Ca2+ from intracellular stores in response to a rise in Ca2+ itself. This process is inhibited by rapamycin, although this drug is best known for its inhibition of mammalian target of rapamycin (mTOR) (see below). DAG, the other metabolite of phosphatidylinositol, functions as a second messenger in cells by activating protein kinase C (PKC), as will be discussed below.
The prostaglandin and leukotriene families of intracellular messengers play important roles in the regulation of signal transduction in the brain and elsewhere. Prostaglandins and leukotrienes are generated by a complex biochemical cascade, depicted in 4–11, initiated by phospholipase A2, which cleaves membrane phospholipids to yield free arachidonic acid. Next, arachidonic acid is cleaved by cyclooxygenase to yield, after numerous enzymatic steps, several types of prostaglandins and other cyclic endoperoxides, such as prostacyclins and thromboxanes, or it is cleaved by lipoxygenase to yield the leukotrienes 4–11.
4–11
Arachidonic acid signaling pathways. A. Arachidonic acid gives rise to many important signaling molecules, which are products of two main pathways. B. One involves 5´-lipoxygenase and gives rise to the leukotrienes. C. The other involves the action of cyclooxygenase and gives rise to three families of signals: prostacyclins D, prostaglandins E, and thromboxanes F. Cyclooxygenases are inhibited by nonsteroidal anti-inflammatory agents. 5,8,11,14-Eicosatetraenoic acid (EA) is a lipoxygenase inhibitor that also inhibits cyclooxygenases.

The varied biologic activities of these endoperoxides and leukotrienes serve to regulate adenylyl cyclase, guanylyl cyclase, ion channels, protein kinases, and other cellular proteins, either directly or by serving as the endogenous ligands for G protein–coupled receptors.
Receptors for the leukotrienes are termed BLT or LT receptors, and those for prostaglandins and thromboxanes are termed prostanoid receptors. Several prostanoid receptors are known, and each is named for the arachidonic acid metabolite with the highest affinity for that receptor. EP receptors, for example, are activated preferentially by prostaglandin E2 (PGE2). All LT receptors are coupled by Gq and presumably act by stimulating the PLC pathway. In contrast, the numerous subtypes of prostanoid receptors are coupled by Gq, Gs, or Gi.
Aspirin, acetaminophen, and all nonsteroidal anti-inflammatory drugs (NSAIDs) such as ibuprofen exert their antipyretic, analgesic, and anti-inflammatory effects by inhibiting cyclooxygenase, which has both constitutive (COX1) and inducible (COX2) forms. COX1 is expressed in many locations, including the upper gastrointestinal tract, where nonselective COX inhibitors block the protective functions of prostaglandins. Such interference increases the risk of peptic ulceration, a common side effect of these drugs. Selective COX2 inhibitors, such as rofecoxib, that are analgesic and anti-inflammatory but with less disturbance of the gastrointestinal tract were in wide use until recent reports of cardiovascular toxicity (Chapter 11).
Classic studies in neuropharmacology assumed that different agonists at a single receptor would, of course, trigger the receptor to signal through its one and only effector pathway, a β-adrenergic receptor through Gs and cAMP, a 5HT2A receptor through Gq and PLC, and so on. Only the agonist’s potency and efficacy would vary, not its qualitative functional effect. In fact, we now know that many receptors can signal through multiple pathways (eg, a β-adrenergic receptor can signal not only through Gs and cAMP but also through arrestin pathways; see 4–3) and that receptors can display qualitatively different signaling effects depending on the agonist. Such ligand-directed or biased signaling, discussed further in Chapters 1 and 6, has greatly complicated efforts to understand drug action, but has also raised the very real possibility of creating novel agonists to a receptor that target one signaling pathway versus another and thus dialing in wanted efficacy while avoiding unwanted side effects.
Most of the effects of intracellular second messengers are produced through their regulation of protein phosphorylation. Protein phosphorylation may inactivate a neurotransmitter receptor, cause an ion channel to open more or less readily, or enable the synthesis of a neurotransmitter much more rapidly. Indeed, virtually every class of neuronal and glial protein is regulated through phosphorylation 4–3, and consequently many prominent forms of CNS plasticity are mediated by such phosphorylation events. Although proteins are covalently modified in many ways—for example, by ADP-ribosylation, acylation, and glycosylation, among many others—none of these mechanisms is as widespread or as readily subject to regulation by synaptic and hormonal stimuli as phosphorylation.
Regulated Protein | Protein Kinase | Effect |
---|---|---|
|
|
|
|
|
|
|
|
|
|
|
|
|
|
|
|
|
|
|
|
|
|
|
|
|
|
|
|
|
|
Protein kinases can be classified according to their sequence homologies 4–4; however, they are presented here based on shared regulatory and functional characteristics. Among the best characterized protein kinases in the brain are those activated by the second messengers cAMP, cGMP, Ca2+, and DAG. All of these protein kinases, named for the second messengers that activate them, phosphorylate substrate proteins on serine or threonine residues, and thus are referred to as protein serine–threonine kinases.
| |
| |
| |
| |
| |
| |
| |
| |
| |
| |
|
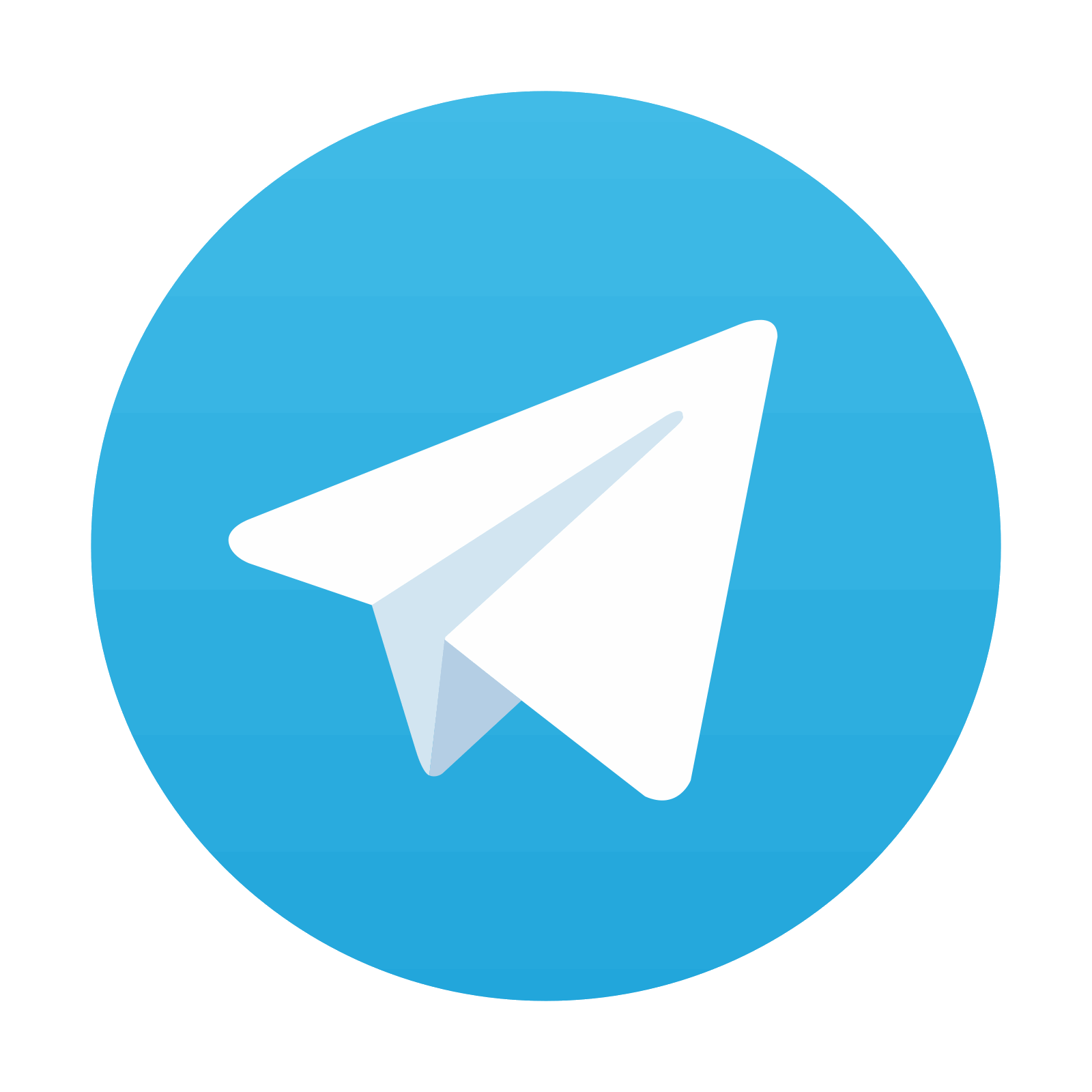
Stay updated, free articles. Join our Telegram channel
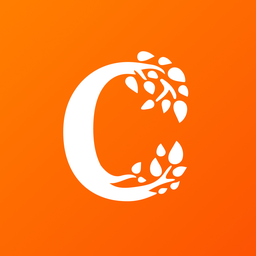
Full access? Get Clinical Tree
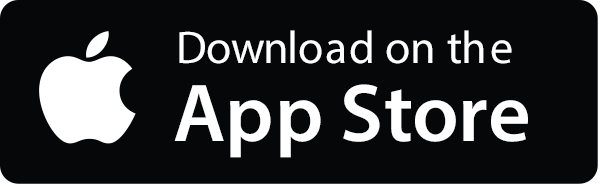
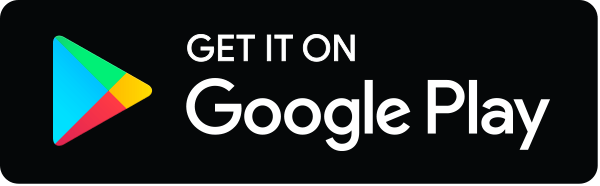
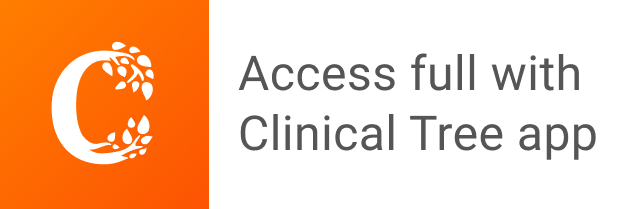