Neurologic Manifestations of Systemic Illness
S. Robert Snodgrass
METABOLIC ENCEPHALOPATHIES
Extracerebral diseases and disturbances may interfere with neurologic function by impairing oxygen and glucose supply or by disturbing the ionic and humoral environment of neurons, glia, and synaptic processes. Neurons and supporting cells require specific chemical environments. Their ability to adapt to such mild deviations from the normal environment as life at high altitudes, elevated body temperature, or decreased serum sodium could hold the key to more effective future treatment for anoxic and metabolic encephalopathies.
Oxygen: Hypoxia and Hyperoxia
Pathophysiology
Hypoxia-ischemia, hypoglycemia, and status epilepticus induce energy failure with consequent brain damage (1,2,3). The significant differences in the time course and distribution of brain damage that result from these three insults are depicted in Table 17.1 and are reviewed by Auer and Sutherland (4). Brain consumes disproportionate amounts of oxygen, has scant glycogen stores, and tolerates hypoxia and hypoglycemia less well than most organs (5). Neurons need constant oxygen and glucose supply to maintain ion and neurotransmitter gradients.
Oxygen pressure is not uniform throughout the brain. It is higher in gray matter than in white matter, as is blood flow and glucose utilization. Brain glucose and oxygen consumption per gram of tissue are higher in neonatal than adult brain (4). The first effect of experimental cerebral hypoxia is an increase of intracellular pH. Subsequently, intracellular calcium content rises as consequence of calcium release from the endoplasmic reticulum. ATP concentration begins to fall, and when 50% to 70% of neuronal ATP is lost, the sodium pump fails and voltage-controlled ion channels open, permitting Na+, K+, Ca++ and Cl– to flow down their concentration gradients and release stored neurotransmitters (6). Water follows because of increased osmolality, and cells swell. Neuronal intracellular calcium concentration can then increase by up to four orders of magnitude. These huge increases in cytosolic [Ca++] activate lipases, proteases, and other catabolic enzymes.
Changes in oxygen pressure have rapid, direct effects on membrane ion channels, mostly phosphorylation-related (7). Some ion channels are down-regulated, reducing ion flux and cellular energy demands (8). Others ion channels are up-regulated, promoting depolarization and cell death (9). Hypoxia also induces a variety of molecules called hypoxia-inducible factors (HIF). HIFs are induced more slowly than the effects on ion channels. They are expressed in all tissues and activate transcription of genes that increase systemic O2 delivery or provide cellular metabolic adaptation under conditions of hypoxia. Thus, they activate the transcription of the gene for erythropoietin, the genes for glycolytic enzymes, and genes involved in angiogenesis. Juul has reviewed the use of erythropoietin to protect against neonatal ischemic brain injury (10); a recent study reported that it decreased brain injury when given to very young rats before hypoxic-ischemic encaphalopathy (HIE) (11). Further investigation is warranted, but the dismal results of human neuroprotective drug trials to date imply that clinical use of these drugs may be far in the future (12). The factors that mediate the induction of HIFs are under intense investigation (13). They are related to the phenomenon of ischemic tolerance, or preconditioning. This term refers to the observation that a brief period of cerebral ischemia confers transient tolerance to a subsequent ischemic insult to the brain (14). The mechanisms underlying ischemic tolerance are not fully understood. Kirino has suggested two possibilities: (a) A cellular defense function against ischemia may be enhanced by the mechanisms inherent to neurons. They may arise by post-translational modification of proteins or by expression of new proteins via a signal transduction system to the nucleus. These cascades of events could strengthen the influence of survival factors or could inhibit apoptosis; (b) a
cellular stress response and synthesis of stress proteins can lead to an increased capacity for health maintenance inside the cell (14). The mechanisms of ischemic tolerance or preconditioning may ultimately become relevant for therapy of HIE (15).
cellular stress response and synthesis of stress proteins can lead to an increased capacity for health maintenance inside the cell (14). The mechanisms of ischemic tolerance or preconditioning may ultimately become relevant for therapy of HIE (15).
TABLE 17.1 Neurobiological Differences Among Ischemia, Hypoglycemia, and Epilepsy | ||||||||||||||||||||||||||||||||||||||||||||||||
---|---|---|---|---|---|---|---|---|---|---|---|---|---|---|---|---|---|---|---|---|---|---|---|---|---|---|---|---|---|---|---|---|---|---|---|---|---|---|---|---|---|---|---|---|---|---|---|---|
|
The mature brain requires both oxygen and glucose but can adapt to reduced serum glucose levels (16). The fetal and neonatal brain also uses ketone bodies as an energy source (17); these metabolic pathways can be increased by diet and fasting (18). In vitro studies show that low glucose media increase hypoxic injury and that this effect correlates well with tissue ATP content (19).
Hypoxic-Ischemic Encephalopathy
HIE as it pertains to neonates is covered in Chapter 6, while localized arterial and venous disease are discussed in Chapter 13.
Hypoxia means reduced blood oxygen content, and ischemia means reduced tissue perfusion. They often go hand in hand because sustained changes in tissue oxygenation have secondary circulatory effects. Asphyxia means impaired gas exchange, as carbon dioxide accumulates as oxygen falls. Mild experimental hypoxemia increases electroencephalogram (EEG) delta activity, prolongs reaction times, and impairs psychological test performance (20).
Acute severe hypoxia impairs consciousness. The duration and severity of hypoxia determines the magnitude of injury and residual impairment, but other factors influence the extent of neural injury. Transient hypoxic episodes may be seen in heart disease, with cardiac arrhythmias, and respiratory problems. Experimentally induced transient hypoxia impairs oculomotor and visual function and can be accompanied by brief nonfocal seizures. These result in no detectable sequelae (21). Stephenson reports “anoxic motor convulsions” with asystole induced by ocular compression (22).
Mild to moderate oxygen desaturation (oxygen saturation in the 80% to 90% range) need not be harmful, especially if brief in duration. The Collaborative Home Infant Monitoring Evaluation (CHIME) study found that 43% of 306 healthy term babies had at least one 20-second episode of apnea or bradycardia without evidence of neurological abnormality (23).
Longer periods of hypoxia produce cellular injury. Neurons are more vulnerable than glial cells to hypoxia and hypoglycemia, and oligodendrocytes are generally more vulnerable than astrocytes (24). Neurons in certain areas of the brain such as the basal ganglia and the hippocampal CA1 pyramidal neurons are particularly vulnerable to asphyxial injury. By contrast, spinal cord neurons tolerate longer periods of occlusion than do cerebral neurons (25). The factors that determine the selective vulnerability of certain neuronal populations are still incompletely understood. They are reviewed in Chapter 6.
How Cells Die
Two types of cell death have been recognized: apoptosis and necrosis. Biologists reported extensive cell death in developing organisms in the 19th century but did not consider cell death to be a central part of normal development (26).
Lockshin’s 1965 PhD thesis on silkworm metamorphosis introduced the phrase “programmed cell death” (PCD) (27). The Australian pathologist John Kerr studied human liver disease and noted an orderly form of cell death characterized by cell shrinkage with nuclear compaction and no inflammation. He first called this shrinkage necrosis (28). Peripheral areas where shrinkage necrosis predominated sometimes surrounded a central zone of coagulation necrosis. Kerr and colleagues later replaced “shrinkage necrosis” with the Greek word apoptosis (29). They saw mitosis and apoptosis as opposing forces, postulating that apoptosis inhibited tumor growth and development. Wyllie reported DNA fragmentation in cells undergoing apoptosis (30). Gel electrophoresis revealed “DNA ladders.” Apoptosis or PCD became a focus of attention, and studies of its cellular control mechanisms in the nematode C. elegans led to a Nobel Prize (31). Vertebrates produce too many neurons; programmed cell death prunes the numbers of neurons and glia. Mice with caspase gene knock-outs (caspases are proteases that trigger some forms of PCD) have gross central nervous system (CNS) malformations (32). Pathologists accepted the idea of qualitatively different forms of cell death, but they initially limited this to two types.
Lockshin’s 1965 PhD thesis on silkworm metamorphosis introduced the phrase “programmed cell death” (PCD) (27). The Australian pathologist John Kerr studied human liver disease and noted an orderly form of cell death characterized by cell shrinkage with nuclear compaction and no inflammation. He first called this shrinkage necrosis (28). Peripheral areas where shrinkage necrosis predominated sometimes surrounded a central zone of coagulation necrosis. Kerr and colleagues later replaced “shrinkage necrosis” with the Greek word apoptosis (29). They saw mitosis and apoptosis as opposing forces, postulating that apoptosis inhibited tumor growth and development. Wyllie reported DNA fragmentation in cells undergoing apoptosis (30). Gel electrophoresis revealed “DNA ladders.” Apoptosis or PCD became a focus of attention, and studies of its cellular control mechanisms in the nematode C. elegans led to a Nobel Prize (31). Vertebrates produce too many neurons; programmed cell death prunes the numbers of neurons and glia. Mice with caspase gene knock-outs (caspases are proteases that trigger some forms of PCD) have gross central nervous system (CNS) malformations (32). Pathologists accepted the idea of qualitatively different forms of cell death, but they initially limited this to two types.
Lucas and Newhouse and Olney defined neuronal cell death caused by excessive glutamate receptor stimulation or “excitotoxicity” (33,34). It was characterized by swelling and bursting of cells, release of lysosomal enzymes, and subsequent inflammation. They called this phenomenon necrosis; any cell may suffer necrosis in the older sense of cell death followed by inflammation; excitotoxic cell death is a special form of necrosis limited to neural tissue with glutamate receptors. Standard teaching about HIE came to include the idea that glutamate leakage from presynaptic stores increased calcium and sodium influx and aggravated the brain injury (35). The characteristics of necrosis and apoptosis are summarized in Table 17.2. Coagulation necrosis refers to the death and disruption of a group of cells and is usually due to ischemia. It can often be detected by magnetic resonance imaging (MRI) in that inflammation and increased water content become visible in the T2 and spin-echo sequences, and contrast enhancement is common, due to breakdown of the blood brain barrier. Apoptosis is not associated with inflammatory changes and is generally invisible to MRI. Its demonstration in the postmortem brain can be difficult because of technical problems and because apoptotic cells are often cleared in minutes or hours, and can disappear within a few days (36). Karyorrhexis can indicate apoptosis but is not specific. The DNA digestion pattern recognized in apoptotic thymocytes by Wyllie gave rise to the TUNEL (terminal deoxynucleotidyl transferase dUTP nick end-labeling) method for identification of apoptosis (37). However, the specificity and sensitivity of the TUNEL method has been increasingly doubted (38), especially in postmortem brain tissue. Kerr defined apoptosis by electron microscopy; this technique is presently the best way to distinguish types of cell death (28). The various forms of PCD have been characterized by Clarke (39) (Table 17.3).
TABLE 17.2 Simple Dichotomy of Necrosis Versus Apoptosis | ||||||||||||||||||||||||
---|---|---|---|---|---|---|---|---|---|---|---|---|---|---|---|---|---|---|---|---|---|---|---|---|
|
Cell death morphology depends upon tissue, cell type, and age of the animal; severity and duration of asphyxia; and the density and characteristics of cellular receptors. These factors are reviewed in Chapter 6. Mixtures of necrosis and PCD may be found in the same section or same cell, with intermediate forms of cell death that include attributes of both PCD and necrosis (40,41). Inhibition of one form of cell death may appear to stimulate another form (42).
Pathologic Anatomy of Cerebral Anoxic-Ischemic Injury
Bakay and Lee (43) and Auer and Siesjö (1) have described the basic pathologic alterations in the hypoxic brain. Structural damage can be limited to neurons or, if the hypoxia is more severe, it also can involve glia and nerve fibers. The microscopic changes in neurons subjected to energy failure have been delineated by Auer and Beneviste (44). As a rule, associated glial cell damage is proportional to neuronal damage. In gray matter, astrocytes swell as a result of cellular overhydration, whereas in white matter, the intercellular space enlarges because of extracellular edema and alterations in the walls of the cerebral capillaries. These pathological alterations are summarized in Table 17.4.
Areas most sensitive to hypoxia, as occurs after sudden cardiac arrest, are the middle cortical layers of the occipital and parietal lobes, the hippocampus, amygdala, caudate nucleus, putamen, anterior and dorsomedial nuclei of the thalamus, and cerebellar Purkinje cells (45). Brainstem nuclei are more likely to be involved in infants than in older children.
Brierley and coworkers have studied the time course of these pathologic changes in adult and juvenile monkeys. The earliest changes seen at the light microscopic level included loss of Nissl substance (ribosomes), eosinophilia, and nuclear changes—the “red dead neurons” (46,47). The nuclear changes may be pyknosis or karyorhexis (fragmentation). Necrosis is accidental and
requires no energy, whereas programmed cell death requires energy-dependent sequential activation of highly conserved proteins. Coagulation necrosis and disruption of tissue architecture—for example, infarction—is expected in the most hypoxic area, with PCD in peripheral areas, where energy supply permits the execution of preprogrammed cell death routines. Experimental stroke studies report caspase-mediated delayed cell death in the region outside maximal injury, with apoptotic features (48). Benjelloun and colleagues found prominent caspase expression in experimental neonatal stroke; it was rare in adults (49). Caspase expression was prominent in neurons and astrocytes in ischemic regions of the neonatal sheep brain (50).
requires no energy, whereas programmed cell death requires energy-dependent sequential activation of highly conserved proteins. Coagulation necrosis and disruption of tissue architecture—for example, infarction—is expected in the most hypoxic area, with PCD in peripheral areas, where energy supply permits the execution of preprogrammed cell death routines. Experimental stroke studies report caspase-mediated delayed cell death in the region outside maximal injury, with apoptotic features (48). Benjelloun and colleagues found prominent caspase expression in experimental neonatal stroke; it was rare in adults (49). Caspase expression was prominent in neurons and astrocytes in ischemic regions of the neonatal sheep brain (50).
TABLE 17.3 Main Types of Cell Death | ||||||||||||||||||||||||||||||||||||||||||||||||
---|---|---|---|---|---|---|---|---|---|---|---|---|---|---|---|---|---|---|---|---|---|---|---|---|---|---|---|---|---|---|---|---|---|---|---|---|---|---|---|---|---|---|---|---|---|---|---|---|
|
TABLE 17.4 Composite Brain Injury Resulting From Neonatal Asphyxia, Sepsis, Cardiac Surgery, and Other Forms of Hypoxia in Small Infants | |
---|---|
|
Cardiac arrest or vascular obstruction of 15 minutes or more in duration is often followed by initially normal or supernormal cerebral blood flow that declines to subnormal values with regions devoid of perfusion. The causes of these progressive changes are unclear; they are common in children resuscitated from drowning or cardiac arrest with initial systemic pH values below 6.9 (51). In part, these changes can be due to increased cerebral vascular resistance with prolonged ischemia. Many other factors can contribute to this increased resistance, including release of intracellular potassium (52), increased blood viscosity, leukocyte margination, and mediators such as bradykinin and nitric oxide. Endothelial changes are often seen with prolonged ischemia and reperfusion (53,54). These processes can contribute to delayed encephalopathy and postanoxic deterioration. They could be part of the cerebral circulatory arrest seen in brain death, and they could be preventable.
Delayed Encephalopathy
The histologic manifestations of anoxic-ischemic brain injury take time to become evident (4,46). Sometimes, there is partial clinical recovery after anoxic events, followed by dramatic clinical deterioration. This syndrome occurs with strangulation (55) and with many forms of anoxia, including near drowning (56,57). It is most dramatic after carbon monoxide poisoning; this phenomenon is considered
in Chapter 10. Most patients with delayed deterioration, whether their initial injury was due to anoxia or carbon monoxide poisoning, develop extensive deep white matter injury—“Grinker’s myelinopathy”(58). However, some patients, such as Dooling and Richardson’s 11-year-old patient (55) and other pediatric cases (59,60) have only gray matter lesions.
in Chapter 10. Most patients with delayed deterioration, whether their initial injury was due to anoxia or carbon monoxide poisoning, develop extensive deep white matter injury—“Grinker’s myelinopathy”(58). However, some patients, such as Dooling and Richardson’s 11-year-old patient (55) and other pediatric cases (59,60) have only gray matter lesions.
Clinical Manifestations
Hypoxia
A number of clinical features are shared by all metabolic encephalopathies (61). The earliest symptom is a gradual impairment of consciousness. In infants, this can take the form of irritability, loss of appetite, and diminished alertness. Periods of hyperpnea can progress to Cheyne-Stokes respiration, a pattern of periodic breathing in which hyperpnea regularly alternates with apnea. The eyes move randomly, but ultimately, as the coma deepens, they come to rest in the forward position.
When anoxia occurs acutely, consciousness is lost within seconds. In cyanotic congenital heart disease, anoxia can take the form of brief syncopal attacks, often after crying, exertion, or eating; most frequently, these occur during the second year of life. Usually, the child cries at the onset of the attack, then becomes deeply cyanotic and gasps for breath. Generalized seizures can terminate the more severe cyanotic episodes.
Should oxygen supply be restored immediately, recovery is quick, but when anoxia lasts longer than 1 to 2 minutes, neurologic signs persist transiently or permanently. These include impaired consciousness and decerebrate or decorticate rigidity. The prognosis for survival is relatively good for patients who after their anoxic episode exhibit intact brainstem function as manifested by normal vestibular responses, normal respiration, intact doll’s eye movements, and pupillary light reactions (61).
The longer the duration of coma, the less likely the outlook for full recovery. In the series of Bell and Hodgson, which included all age groups, 17.5% of patients comatose for longer than 24 hours could be discharged from the hospital, but 70% of these subjects experienced significant and permanent neurologic impairment (62). There is fairly good evidence that some children who survive a major hypoxic episode without apparent gross neurologic residua are left with permanent visuoperceptual deficits (63).
The EEG is of assistance in predicting the outcome of coma after cardiorespiratory arrest. A phasic tracing early in the recovery period indicates a good prognosis, whereas a flat EEG is never associated with full recovery other than in cases of drug ingestion (64). Bilateral loss of cortical responses after median nerve stimulation on the somatosensory-evoked potential (SSEP) test is one of the best prognosticators for a poor outcome. Initial preservation of the cortical potentials does, however, not necessarily imply a good recovery (65,66). This is particularly true for small infants, and serial SSEPs are indicated to ascertain whether they continue to remain intact (67). In term neonates, the positive predictive value of an abnormal SSEP also is excellent, but in premature infants, a normal response after stimulation of the median nerve had a poor predictive value with respect to normal outcome (67,68). As a rule, evoked potentials, specifically somatosensory-evoked potentials, are much more useful indicators of prognosis in postanoxic coma than is the EEG (69). The outcome of pediatric patients resuscitated from cardiac arrest is reviewed in Table 17.5 (70,71).
TABLE 17.5 Combined Results of Two Controlled Trials of Hypothermia as Treatment for Adults Who Had Out-of-Hospital Cardiac Arrest | |||||||||||||||||||||||||||
---|---|---|---|---|---|---|---|---|---|---|---|---|---|---|---|---|---|---|---|---|---|---|---|---|---|---|---|
|
Near Drowning
In near drowning, the length of coma has even more significant prognostic implications than after cardiorespiratory arrest, and, as a rule, there is an all-or-nothing outcome, with few children experiencing mild degrees of neurologic damage. None of the patients still comatose in 15 to 30 minutes after their rescue survived without major neurologic residua, and 60% of subjects in this group died. In a Hawaiian series, all children who ultimately survived intact made spontaneous respiratory efforts within 5 minutes of rescue, and the majority of those did so within 2 minutes (72). The experiences from several other centers are similar in that all children who still required cardiopulmonary resuscitation on arrival at the hospital experienced permanent severe anoxic encephalopathy. Interestingly, the presence of convulsions does not indicate a bad prognosis, although their persistence beyond 12 hours does. However, the appearance of myoclonic status epilepticus after cardiac arrest is a poor prognostic sign (73). Fields lists the following factors that predict poor outcome: (a)
submersion for more than 5 minutes; (b) serum pH below 7.0 at time of admission to the emergency room; (c) the need for cardiopulmonary resuscitation in the emergency room; (d) a delay before the first postresuscitation gasp; and (e) poor initial neurologic evaluation on resuscitation (74). Immersion in cold or icy water appears to give a better chance for survival (75).
submersion for more than 5 minutes; (b) serum pH below 7.0 at time of admission to the emergency room; (c) the need for cardiopulmonary resuscitation in the emergency room; (d) a delay before the first postresuscitation gasp; and (e) poor initial neurologic evaluation on resuscitation (74). Immersion in cold or icy water appears to give a better chance for survival (75).
SSEPs and an EEG obtained during the second 24 hours after the accident have been used as additional prognostic indicators (76).
A postanoxic dystonic syndrome has been recognized in children. It appears 1 week to 36 months after the anoxic insult and tends to worsen for several years. Dysarthria and dysphagia are common. Neuroimaging studies reveal putaminal lesions in the majority of such cases. Treatment is generally ineffectual. The pathophysiologic mechanism underlying this condition and the reason for its progression are totally unknown (77).
The persistent vegetative state (PVS) after near drowning is being seen with increasing frequency owing to the resuscitative facilities of most emergency rooms. According to data compiled in California and reported in 1994, survival of children in PVS is dependent on their age. Median survival of infants younger than 1 year of age was 2.6 years; of infants between 1 and 2 years, 4.2 years; and children between 2 and 6 years, 5.2 years (78). In 1999, it was found that in the mid-1990s, the mortality rate for infants in PVS was only one-third of that in the early 1980s. A smaller decrease in mortality rates was recorded for children ages 2 to 10 years (79). In the experience of Heindl and Laub, 55% of children who were in PVS as a result of an anoxic event became conscious within 19 months of the injury. The quality of life was fairly good for those who recovered from PVS; 9% recovered completely, and another 52% became independent in everyday life (80). After 9 months, less than 5% of children were able to recover from PVS (80). In the study of Ashwal and coworkers (78), children in PVS survive somewhat longer in institutions than at home; other studies have shown converse results (81). A consideration of PVS following head trauma can be found in Chapter 9.
Treatment
The goals of treatment for cardiac arrest and postanoxic coma whatever its cause are to stabilize cardiac function, prevent further brain injury including delayed encephalopathy, and to maximize recovery of function. Cardiac arrest typically occurs at unexpected and inopportune times. Therefore, advance protocols or treatment outlines are desirable. Numerous treatment regimens have been used for cerebral salvage. These include induced hypothermia, barbiturate coma, and intracranial pressure monitoring to control cytotoxic cerebral edema. None of these has been effective in improving the ultimate outcome (75,85). The neurologist attending a near-drowning victim should keep in mind that hypoglycemia and hyperglycemia can cause further neurologic damage. Hyperthermia should be avoided and seizures controlled, with phenytoin being the preferred anticonvulsant. Animal studies suggest that vasopressin may be superior to epinephrine for resuscitation after cardiac arrest, and some suggest using both drugs (86). However, a human study failed to show a clear difference in outcome when either vasopressin or epinephrine was used (87). A study of 68 pediatric patients found that high-dose epinephrine was no better than and possibly inferior to standard-dose epinephrine given after an initial unsuccessful standard epinephrine dose (88). Neither magnesium sulfate nor diazepam given after out-of-hospital resuscitation improved outcome (89). Moderate hypothermia (12 to 24 hr at 32° to 34°C) improved outcome of comatose survivors of out-of-hospital cardiac arrest in two small European studies (90,91). The details associated with hypothermia are important—such as use of paralysis and sedation and possible interaction between hypothermia and various other drugs. Volume restriction is generally unwise; Ginsberg (92) advises the use of albumin infusions not only to increase plasma volume and decrease blood viscosity (93), but also to bind fatty acids and other toxins. Glucocorticoids worsen the effects of HIE in vivo and oxygen-glucose deprivation in vitro in that they induce hyperinsulinemia and hyperglycemia and have complex effects on glucose transport and energy metabolism (94,95). They also impair neuronal and astrocytic glucose uptake and inhibit astrocytic uptake of excitatory amino acids promiscuously released during anoxic insults (96).
Although there are no current standards of practice or uniformly accepted regimens at this time, I recommend the following:
Hypothermia to 32.5° to 33°C for 24 to 36 hours starting soon after arrival in the pediatric intensive care unit (PICU).
Full fluid maintenance, unless there is renal, hepatic, or cardiac failure. In prior years, clinicians restricted the use of intravenous (IV) fluids, hoping to avoid cerebral edema. This encouraged sludging in cerebral microvessels and had little effect on the occurrence or severity of cerebral edema. Hypotonic fluid should be avoided. I use albumin if there is any reason to suspect that the patient has been volume depleted. Sometimes, I use full maintenance fluids with colloid and a loop diuretic.
Unless there is GI bleeding, I give rectal aspirin once daily, 5 to 7 mg/kg for the first three days plus an H2 blocker to reduce gastric acid secretion until feeding begins. Celecoxib has been beneficial in experimental studies (97), but the selective COX-2 inhibitors appear to be more thrombogenic than aspirin, and I believe that aspirin antiplatelet actions are beneficial.
I resume ventilation with room air as soon as feasible—but if there is lung disease or arterial hypoxemia, I continue high oxygen ventilation because of the oxidative stress that it imposes. I urge avoidance of sustained hyperventilation, which may increase cerebral ischemia (98).
Use anticonvulsants or glucocorticoids only if there is a specific indication other than anoxic brain injury. Most and possibly all antiepileptic drugs cause apoptosis and death of neurons in very young rats (99). This risk in humans is probably greatest for infants less than 2 years old. I use antiepileptic drugs if there are clinical seizures. It is debatable as to whether treatment of electrographic seizures without clinical manifestations is helpful in hypoxic coma.
It is important to avoid hyperglycemia, which makes cerebral ischemia worse and increases edema in experimental brain hemorrhage (100).
Brain Death
The concept of brain death developed once it became possible to maintain vital functions in patients with massive brain injury. Studies during the 1960s suggested that patients without evidence of brain electrical activity did not recover unless their condition was due to drug overdose. However, those studies included few children and no neonates.
In 1987, the Ad Hoc Committee on Brain Death from the Children’s Hospital, Boston, defined brain death as follows:
Brain death has occurred when cerebral and brainstem functions are irreversibly absent. Absent cerebral function is recognized clinically as the lack of receptivity and responsivity, that is, no autonomic or somatic response to any sort of external stimulation, mediated through the brainstem. Absent brainstem function is recognized clinically when pupillary and respiratory reflexes are irreversibly absent…. Particularly in children, peripheral nervous activity, including spinal cord reflexes, may persist after brain death; however, decorticate or decerebrate posturing is inconsistent with brain death (101).
Recommendations made in 1987 by a special task force appointed to set guidelines for determining brain death in children have been published (102). Although it is generally recognized that particular caution should be exerted when diagnosing brain death in small children, the task force further emphasized this age distinction by recommending different brain death criteria for infants between 7 days and 2 months of age, between 2 months and 1 year, and those older than 1 year. The period of observation before declaration of brain death in the youngest group should be such that two examinations and EEGs to document electrocerebral silence are performed, separated by at least 48 hours. In the group from 2 months to 1 year of age, the interval between the two examinations and EEGs can be reduced to 24 hours. The clinical examination of the pediatric patient suspected of being brain dead is summarized in Table 17.6 (103,104,105). A repeat examination and EEG are not necessary in this group if radionuclide angiography demonstrates absent cerebral blood flow (106). In children older than 1 year, the task force recommended the period of observation be a minimum of 12 hours, unless corroborating tests added further support to the diagnosis of brain death. When the extent and reversibility of brain damage are difficult to assess because of the type of insult (e.g., hypoxic-ischemic encephalopathy), the observation period should be extended to at least 24 hours. The confounding problem today is that most children die in PICUs and receive many medications in the last days of life. It is likely that “therapeutic and falling” levels of barbiturates and other anticonvulsants do not invalidate ordinary clinical and EEG criteria for brain death (107), but drug metabolism can be very slow in the brain dead patient, and high levels of other drugs such as opioids are often present. EEG and evoked potentials may occasionally become unobtainable, only to return hours or days later (108).
TABLE 17.6 Clinical Evaluation of the Possibly Brain Dead Patient | ||
---|---|---|
|
An important aspect in diagnosing brain death is the documentation of apnea. During this procedure, it is vital to prevent hypoxemia. Administration of 100% oxygen for 10 minutes is recommended before withdrawal of respiratory support. A catheter should be inserted into the endotracheal or tracheostomy tube, and oxygen be continued at 6 L per minute during the test. The arterial pCO2 level
should be allowed to increase to 60 mm Hg. Patients who are hypothermic or receiving medications that suppress respiration cannot be reliably tested using this procedure (70).
should be allowed to increase to 60 mm Hg. Patients who are hypothermic or receiving medications that suppress respiration cannot be reliably tested using this procedure (70).
Some authorities have therefore challenged these recommendations, and a survey of PICUs shows substantial variability, even within the same unit, with respect to criteria used by clinicians for the diagnosis of brain death (109). In a clinical and neuropathologic study of brain death, Fackler and coworkers found no support for employing distinct brain death criteria for infants between 2 months and 1 year of age (110). Other investigators question the validity of relying on the EEG to confirm brain death because EEG activity is occasionally seen after brain death (111). Conversely, phenobarbital levels above 25 to 35 μL can suppress EEG activity in neonates (112). The brainstem auditory-evoked response cannot be used as a confirmatory laboratory criterion of brain death. Its absence is not predictive of brain death, and persistence of peak I has occasionally been seen in brain dead infants (113).
Demonstration of cerebral circulatory arrest is the most convincing proof of brain death and is not influenced by drugs or hypothermia. In principle, this can be done by transcranial Doppler ultrasonography in the hands of an experienced technician (114), by radionuclide angiography (115), or by spiral CT (116). However, these methods are less precise than angiography or MRI. Ruiz-Garcia and colleagues compared EEG, radionuclide angiography, and evoked potentials in 125 brain dead Mexican children. There was considerable concordance, but only 61 of 76 children who were subjected to all three studies had positive results on all three (115). Although complete absence of cerebral blood flow is considered irrefutable evidence of brain death, it is necessary to consider that cerebral blood flow is extremely low in normal term or preterm newborns (117).
Although CT angiography (118) and MRI and MR angiography (119) offer even more precise demonstration of cerebral circulatory arrest, they require moving the patient to the radiology suite. High-resolution brain CT scans with contrast are quite satisfactory for this purpose. If there are no flow voids in a technically satisfactory MRI of the brain, the patient is brain dead.
I believe that as these more sophisticated imaging techniques are applied to the clinical evaluation of brain death in children, the criteria for making this diagnosis will become refined and perhaps simplified.
Hyperoxia
Hyperoxia is iatrogenic, the result of increased oxygen content of inspired air or hyperbaric oxygen therapy. The question is when and how hyperoxia under normal atmospheric pressure causes neuronal or glial cell death. Hyperoxia is well known to cause cell death in the eye and lung. Hu and coworkers found that both hypoxia and hyperoxia caused cell death with apoptotic features in 7-day-old rat cerebral cortex; hyperoxia after hypoxia did not prevent cell death (120). Several animal studies show mild deleterious effects of normobaric hyperoxia used for resuscitation, and hyperbaric oxygen treatment can cause seizures and permanent brain injury in adults (121,122). Children breathing 100% oxygen develop hyperintense cerebrospinal (CSF) signs on FLAIR (FLuid Attenuated Inversion Recovery) sequences. These partially or completely disappeared when FiO2 was reduced to 30% (123). The controversies with respect to the value of oxygen in resuscitation have been reviewed by Saugstad (124).
Disorders of Glucose Homeostasis
Glucose is necessary for neuronal function. Lactate, pyruvate, and ketone bodies can partially support brain energy needs, but there is always a requirement for some glucose supply. Brain glucose content is less than blood glucose content and increases only slightly with hyperglycemia (125). This is because the delivery of glucose, lactate, and ketone bodies to the brain requires specific transporters, glucose and monocarboxylic acid transporter proteins (GLUTs and MCTs), respectively, and the number of transporter molecules available limits glucose penetration into cells. Neonates have less than half as many transporters per gram of brain tissue as adults (126). GLUT1 is located at the blood–brain barrier and GLUT3 at neuronal membranes (127). GLUT1 is the most widely distributed glucose transporter, with some expression being found in almost every organ. Mutations of GLUT1 produce a progressive encephalopathy with seizures appearing early in life, a condition covered in Chapter 1.
Hypoglycemia
Pathology
Many authorities state that hypoglycemia produces a different topography of cerebral lesions than HIE, in that gray matter is predominantly affected (4,128). There are many exceptions to this dictum, and brain imaging studies often show white matter and thalamic lesions associated with neonatal hypoglycemia (129,130,131). These lesions tend to resolve with prompt therapy (131).
Anatomical studies disclose a selective neuronal necrosis of the superficial cortical layers, the hippocampus, and dentate gyrus. The cerebral cortical lesions are most conspicuous in the insular and the parieto-occipital cortices (132). The thalamus and non-neuronal elements are spared unless hypoglycemia is severe and prolonged (133,134,135). Damage to Purkinje cells is less than occurs after hypoxia (4,136). Infarction or hemorrhage are usually absent, even after a severe hypoglycemic insult (137). As occurs in hypoxia, the accumulation of excitatory neurotransmitters plays an important pathogenetic role in neuronal damage
and death (137). The predominant release of aspartate into extracellular fluid in response to hypoglycemia contrasts with the release of glutamate in hypoxia and may account for the differences in the distribution of neuronal damage. The presence of acidosis, as occurs in hypercapnia, aggravates hypoglycemic neuronal damage, as does concurrent hypoxia (136,138).
and death (137). The predominant release of aspartate into extracellular fluid in response to hypoglycemia contrasts with the release of glutamate in hypoxia and may account for the differences in the distribution of neuronal damage. The presence of acidosis, as occurs in hypercapnia, aggravates hypoglycemic neuronal damage, as does concurrent hypoxia (136,138).
Clinical Manifestations
The clinical manifestations of neonatal hypoglycemia are distinct from those developing at a later stage in life. In a landmark paper published in 1937, Hartmann and Jaudon identified symptomatic hypoglycemia in a group of babies from St. Louis Children’s Hospital (139). They pointed to symptoms of tetany, twitching, convulsion, sweating, and irregular respiration as signs of hypoglycemia. They noted that normal newborns had lower glucose values than adults and older children during the first days of life and reported 12 infants with “cryptogenic hypoglycemia” values below 50 mg/dL without symptoms. Infants of diabetic mothers were recognized as being especially prone to hypoglycemia (140).
The incidence of neonatal hypoglycemia is uncertain because the lower limit of normal for asymptomatic babies is open to dispute (141). Furthermore, we are still unsure whether asymptomatic hypoglycemia is harmful to newborns (142,143).
Symptoms of hypoglycemia in the neonate are nonspecific. Table 17.7 outlines the clinical picture of symptomatic hypoglycemia in term neonates, as recorded from a Finnish nursery (144).
Transient hypoglycemia has been observed in a relatively significant proportion of infants with intrauterine growth retardation, perinatal asphyxia, or other forms of perinatal stress (145,146) and in neonates born to mothers with diabetes or toxemia (147). The incidence of neonatal hypoglycemia is difficult to ascertain because of the different criteria used to define hypoglycemia as well as because of the varieties of feeding routines used in nurseries. Normal plasma glucose values during the first week of life have been published (148). With hypoglycemia defined as glucose levels of 20 mg/dL or less, the condition was identified in 5.7% of cases at the University of Illinois Hospital nursery (149). The incidence is higher in low-birth-weight infants than in term infants.
TABLE 17.7 Symptoms of Neonatal Hypoglycemia in 44 Newborn Patientsa | ||||||||||||||
---|---|---|---|---|---|---|---|---|---|---|---|---|---|---|
|
Symptoms of hypoglycemia may appear as early as 1 hour after birth, particularly in infants who are small for gestational age, but generally they are delayed until 3 to 24 hours. In approximately 25%, hypoglycemia does not become symptomatic until after 24 hours (144). An inconstant relationship exists between blood glucose levels and hypoglycemic symptoms. Some infants with blood sugar levels between 20 and 30 mg/dL develop hypoglycemic symptoms, whereas others whose levels fall below 20 mg/dL can remain asymptomatic (150). Hypothermia can be a useful sign of hypoglycemia. Tonic postures, chorea, and other movement disorders are occasionally seen during hypoglycemia. In rare cases, they become permanent after multiple hypoglycemic episodes (151). Recurrent hypoglycemia is more dangerous than is a single episode (152).
Evoked potentials have provided some evidence to suggest the critical value at which hypoglycemia affects the brain. Somatosensory-evoked potentials (SSEP) and brainstem auditory-evoked potentials (BAER) become abnormal in term infants when their blood sugar falls below 41.5 and 45.0 mg/dL, respectively (153). Visual-evoked responses remain normal at these levels. Koh and coworkers studied BAERs and SSEPs in 17 children during normal and subnormal glucose values (153). Only five of these children were less than 1 week old, two were teenagers, and 13 were hospitalized for investigation of possible endocrine or metabolic disorders. Many showed prolonged brain stem conduction (increased interval between waves I–V in the BAER) when blood sugar fell below 2.6 mmol/L (46 mg/dL). Data from this small and selected group without analogous studies on asymptomatic neonates cannot firmly establish normal or safe blood glucose values (154). From the point of view of a neurologist, it therefore seems prudent that any blood glucose value of 45 mg/dL or less should be emergently corrected and followed closely to ensure sustained normoglycemia.
A rapid compensatory increase in cerebral blood flow resulting from recruitment of previously unperfused capillaries mediated by an increase in plasma epinephrine levels occurs at or below blood glucose values of 30 mg/dL (155,156,157).
The clinical management of hypoglycemia in neonates is beyond the scope of this text. The reader is referred to a flow diagram by Cornblath and Schwartz (158).
It is difficult to know what the outlook is in terms of neurologic and cognitive deficits for neonates who develop symptomatic hypoglycemia. This is because of limitations of current definitions for neonatal hypoglycemia, our inability to determine at what glucose level hypoglycemia
becomes symptomatic, and various other risk factors, which complicate the clinical course of hypoglycemic infants and confound every study on neurodevelopmental outcome (159). From a multitude of data derived from neonates without other major risk factors, who had severe hypoglycemia as a consequence of nesidioblastosis, it is clear that a significantly low plasma glucose level that persists over a prolonged period of time can indeed result in major brain damage. The risks of asymptomatic neonatal hypoglycemia are even more undefined because low-birth-weight and stressed infants, the group with the highest incidence of hypoglycemia, also are subject to a variety of other prenatal and perinatal risks, notably hypoxic ischemic encephalopathy (160). It is prudent to assume that blood sugars below 30 mg/dL can be harmful and should be treated in neonates. A higher critical value of 45 mg/dL or below is often cited for older children but is probably too high, since children placed on the ketogenic diet often have values much less than this without symptoms (161).
becomes symptomatic, and various other risk factors, which complicate the clinical course of hypoglycemic infants and confound every study on neurodevelopmental outcome (159). From a multitude of data derived from neonates without other major risk factors, who had severe hypoglycemia as a consequence of nesidioblastosis, it is clear that a significantly low plasma glucose level that persists over a prolonged period of time can indeed result in major brain damage. The risks of asymptomatic neonatal hypoglycemia are even more undefined because low-birth-weight and stressed infants, the group with the highest incidence of hypoglycemia, also are subject to a variety of other prenatal and perinatal risks, notably hypoxic ischemic encephalopathy (160). It is prudent to assume that blood sugars below 30 mg/dL can be harmful and should be treated in neonates. A higher critical value of 45 mg/dL or below is often cited for older children but is probably too high, since children placed on the ketogenic diet often have values much less than this without symptoms (161).
When older infants and children develop symptomatic hypoglycemia, the condition presents with autonomic symptoms, which accompany a progressive impairment of neurologic function. The serum glucose level at which symptoms appear varies, but any child with a blood glucose level of 46 mg/dL or less is suspect for symptomatic hypoglycemia (162). Autonomic symptoms are mainly caused by increased adrenaline secretion. They include anxiety, palpitations, pallor, sweating, irritability, and tremors (162). During the initial stages, impaired neurologic function is manifested by dizziness, headache, blurred vision, somnolence, and slowed intellectual activity. Transient cortical blindness is seen only rarely (163). In fact, if permanent blindness accompanies hypoglycemia, one must consider the diagnosis of congenital optic nerve hypoplasia associated with hypopituitarism (164). If hypoglycemia is prolonged, subcortical and diencephalic centers become inoperative. The brainstem, the area most resistant to hypoglycemia, is the last to be affected.
Almost all children develop generalized or focal seizures during a severe hypoglycemic episode. With even more prolonged involvement, tonic extensor spasms and shallow respirations develop. The response to intravenous glucose is immediate in patients who have not progressed to brain stem involvement. In children who have experienced prolonged unconsciousness or repeated hypoglycemic attacks, the prognosis for complete recovery is poor, and approximately one-half of patients remain mentally retarded (165).
Not uncommonly, the clinician encounters a child whose first seizure occurred in the setting of suspected hypoglycemia, but who continues to experience seizures in the absence of hypoglycemia. Although prolonged hypoglycemia can indeed induce hippocampal damage and thus set up a seizure focus, I believe that isolated hippocampal damage is quite rare and that, in the majority of such cases, both initial and subsequent seizures are unrelated to hypoglycemia. Transient hemiparesis or aphasia has been seen in diabetic children, often in association with documented hypoglycemia. The cause of these focal deficits is unclear, but they could reflect focal seizures followed by Todd’s paralysis (166).
Hyperglycemia
Hyperglycemia aggravates ischemic brain injury. Rats made hyperglycemic before cerebral ischemia have greater brain damage and mortality (167). This is understandable from Myers’s studies showing that feeding prior to cardiac arrest resulted in postischemic lactic acidosis (168). Even if hyperglycemia is induced after the ischemic period, recovery of function is impaired compared with normoglycemic controls (169). Therefore, normoglycemia is aimed for in acutely ill patients and patients undergoing cardiac surgery. In adult patients, hemiparesis, confusion, and movement disorders may be seen with either hypoglycemia or hyperglycemia (170).
Thermal Stress: Hyperthermia and Hypothermia
Increased body temperature is common in children, usually caused by infections. Children occasionally develop hyperthermia because of anesthesia-related malignant hyperthermia, drug effects, when sweating is impaired by extensive body casts and braces, or when children are left inside vehicles or covered in bed during hot weather (171). Children are more susceptible to heat illness than adults for many reasons, including a greater surface area to body mass ratio, a lower rate of sweating, and a slower rate of acclimatization. The drugs most often contributing to hyperthermia are cocaine and anticholinergic drugs. Life-threatening hyperthermia is less common; it can be seen with neuroleptic malignant syndrome (172), malignant hyperthermia (173), cocaine ingestion (174), and baclofen withdrawal (175). Baclofen withdrawal presents special problems because most such patients have intrathecal baclofen pumps that fail (176). The first sign of pump failure may be severe fever, tachycardia, and hypertension. There is no IV form of baclofen, but I have given such patients fluids, benzodiazepines, rectal baclofen, and cyproheptadine (177). Cocaine-induced hyperthermia is generally associated with exercise and/or warm ambient temperatures. Cocaine impairs sweating and cutaneous vasodilatation. It shares with Ecstasy and several other drugs the property of impairing subjective perception of overheating and need for fluids (174,178,179). Other drugs that can induce hyperthermia include zonisamide (180), topiramate (181), and less commonly, acetazolamide. Serotonin selective reuptake inhibitors (SSRIs) cause the serotonin syndrome,
which can be associated with fever and sweating (182) but rarely induce renal failure and collapse.
which can be associated with fever and sweating (182) but rarely induce renal failure and collapse.
The prevention of heat illness is based on recognizing and modifying risk factors, which include environmental conditions, clothing, hydration, and acclimatization.
Encephalopathy is prominent early in the course of heat injury and is required for the diagnosis of heat stroke. It may progress to renal failure and multiple organ dysfunction with disseminated intravascular coagulation unless vigorously treated (183). Bytomski and Squire (179) cover special issues related to children.
Hypothermia and Cold Injury
Infants are more susceptible to hypothermia than older children. As body temperature falls progressively, many complications ensue (184,185). Electrolyte abnormalities and disseminated intravascular coagulation are common in both sustained hyper- and hypothermia (186). Hypokalemia is the most common electrolyte abnormality seen with hypothermia and represents a shift of potassium into cells. If extra potassium is given, severe complications may arise when the patient is rewarmed (187). Hypothermia accompanied by vomiting and coma are common manifestations of alcohol intoxication in prepubertal children in cold climates (188). Several rare syndromes of periodic hypothermia exist (189,190), including periodic hypothermia and hyperhidrosis, sometimes associated with callosal abnormalities and called Shapiro’s syndrome (191). These periodic diencephalic disorders may respond to clonidine or cyproheptadine and may be related to migraine (192).
Disorders of Acid–Base Metabolism
Brain function and excitability are pH sensitive. The pH of body fluids is tightly regulated, and permeability barriers separate the central nervous system from body fluids. Those barriers are more permeable to carbon dioxide than to protons. Brain extracellular fluid contains more protons and free or total Mg++ ions and less potassium than plasma; the intracellular compartments of neurons and astrocytes are quite different. The brain extracellular environment is regulated or programmed to contain more H+ than plasma or most bodily fluids. This relative acidity of the CSF and brain interstitial fluid is due to metabolic acid production (193).
Many voltage-gated ion channels in the nervous system are pH sensitive. Falling pH (acidosis) inhibits voltage-gated ion channels and glutamate-activated ion channels such as NMDA receptors (194,195). Since voltage-regulated calcium and sodium channels are more sensitive to pH than are potassium channels, increasing pH (alkalosis) increases calcium and sodium entry into neurons, making them more excitable. Acute metabolic disturbances, whether primarily pH changes or changes in electrolyte content of body fluids, often cause seizures and disturbances of consciousness. Respiratory alkalosis may have various causes, including liver disease and acute respiratory distress syndrome (ARDS), and may aggravate seizures from any cause. Respiratory alkalosis is more likely to increase glutamate release than metabolic alkalosis. In general, CSF pH fluctuates less than arterial pH and is well maintained unless changes are acute and severe. The neurological component of acid–base disturbances is nonspecific and poorly correlated with blood or spinal fluid pH.
Electrolyte Disorders
Sodium
Hyponatremia
Sodium chloride (NaCl) is responsible for the largest fraction of osmoles in body fluids, except for cochlear endolymph, a most unusual fluid whose sodium–potassium ratio is about 0.01. Changes in plasma sodium concentration are the first and most obvious sign of water intoxication or water deficiency. Brain extracellular fluid is normally isotonic with plasma. If plasma osmolarity changes rapidly, the brain behaves like an osmometer—it swells when plasma osmolarity decreases and shrinks when plasma osmolarity rises due to water loss. Both hyponatremia and hypernatremia disturb CNS function by changing the osmolality of brain cells. The difference between osmolarity and osmolality is that the former refers to concentration per liter of solution (plasma or CSF), while the latter refers to concentrations per liter of solvent (in this case, water). The reader is referred to a review by Katzman and Pappius (196) for a full discussion of the pathogenesis of cerebral symptoms in electrolyte disorders and to a review by Strange on disorders of osmotic balance (197).
Hyponatremia
Low-sodium syndromes can result from an increase in body water with retention of a normal sodium store or can occur after reduction of sodium stores. It is the most common electrolyte disturbance, occurring in about 2.5% of hospitalized patients, and is even more frequent in neurological and neurosurgical inpatients (198). Hyponatremia is frequently associated with cerebral edema as well as with an increased mortality rate (199).
In the experience of Arieff and colleagues, the most common cause for symptomatic hyponatremia in the pediatric population was administration of hypotonic fluids combined with extensive extrarenal loss of electrolyte-containing fluids (199). Oral water intoxication from increased intake of tap water during the summer months also induces symptomatic hyponatremia (200). Table 17.8
lists the major causes of salt loss in children, and Table 17.9 lists the clinical presentation of hyponatremia in children with CNS disease in the experience of Bussmann and colleagues (201).
lists the major causes of salt loss in children, and Table 17.9 lists the clinical presentation of hyponatremia in children with CNS disease in the experience of Bussmann and colleagues (201).
TABLE 17.8 Clinical Conditions Producing Abnormalities of Sodium Concentration | |
---|---|
|
Neurologic symptoms of hyponatremia include headache, nausea, incoordination, delirium, and, ultimately, generalized or focal seizures with apnea and opisthotonus (202,203). On autopsy, cerebral edema and transtentorial herniation are seen (199,200).
Generally, severe neurologic symptoms with permanent residua do not develop at sodium levels above 130 mEq/L, unless plasma sodium has decreased rapidly. Some have advocated rapid correction of hyponatremia in a patient with neurologic symptoms using urea in conjunction with salt supplements and water restriction (204).
TABLE 17.9 Hyponatremia in Children with Central Nervous System Disease | ||||||||||||||||
---|---|---|---|---|---|---|---|---|---|---|---|---|---|---|---|---|
|
Central pontine myelinolysis was first reported in alcoholics in 1959. It is a frequently fatal disorder that typically appears after major encephalopathy has begun to improve. Clinically, it is characterized by confusion, cranial nerve dysfunction, and in larger lesions, a “locked in” syndrome and quadriparesis. Pathologically, central pontine myelinolysis is marked by symmetric destruction of myelin at the center of the pons. The pontile demyelination can be visualized by MRI (205). It has been associated with rapid correction of hyponatremia, although the exact rate of correction that is safe or dangerous is debated (206). According to Brunner and colleagues, central pontine myelinolysis is more likely to develop when the initial sodium level is less than 105 mEq/L, when hyponatremia has developed acutely, and when sodium levels are corrected too rapidly (207). Myelinolysis is not specific for hyponatremia. It may involve other structures in addition to the pons (207) and may be seen with correction rates of less than 12 meq/L per day (208).
Salt retention rather than salt loss occurs in the syndrome of inappropriate antidiuretic hormone (SIADH) secretion (209). Hyponatremia in the presence of neurologic disorder should not automatically be ascribed to the SIADH (201). It is often due to the syndrome of cerebral salt wasting (CSW). Both of these syndromes tend to begin within 1 or 2 days of the neurologic insult. Table 17.10 reviews the distinction between hyponatremia due to SIADH and that due to CSW.
Setting aside these two syndromes common in patients with neurologic disorders, hyponatremia is generally caused by water retention, as in congestive heart failure, excessive water intake, or loss of electrolytes in excess of water due to gastrointestinal (GI) or renal disease. Many psychotic patients drink excessive amounts of water, sometimes to the point of causing death (210). Hyponatremia also may be caused by excessive water intake in hot weather or after exercise (200). It is occasionally seen in children taking carbamazepine or oxcarbazepine, but few of those children have serum Na+ values below 132 mEQ/L. In general, hyponatremia is not clinically significant until sodium falls below this level, with some exceptions if it develops rapidly (211).
Hypernatremia
Increased concentration of sodium in body fluids elevates fluid osmolality and induces severe cerebral manifestations. Major causes for hypernatremia are outlined in Table 17.8.
Luttrell and Finberg have delineated the factors responsible for neurologic symptoms. These are subdural hematomas, venous and capillary congestion, and hemorrhages, the last produced by shrinkage of the brain during dehydration (212).
Neurologic symptoms also can occur in the absence of any alterations in brain structure and are probably the direct result of hyperosmolality. Symptoms are caused by
cerebral edema, which is particularly likely to occur with rapid rehydration and is caused by an elevated content of chloride and potassium in the brain (213,214).
cerebral edema, which is particularly likely to occur with rapid rehydration and is caused by an elevated content of chloride and potassium in the brain (213,214).
TABLE 17.10 Differences Between the Syndrome of Inappropriate Antidiuretic Hormone Secretion and Cerebral Salt Wasting Syndrome | ||||||||||||||||||||||||
---|---|---|---|---|---|---|---|---|---|---|---|---|---|---|---|---|---|---|---|---|---|---|---|---|
|
Hypernatremia is generally seen in infants younger than 6 months of age. Dunn and Butt compared 57 Australian pediatric inpatients with marked hypernatremia (Na+ >165) to children with hyponatremia (Na+ <115). The sodium disturbance developed after hospitalization in more than half, indicating management failures (215). Hypernatremic patients were more likely to have neurologic symptoms (79%) and death (37%) than hyponatremic children, of whom 58% had neurologic symptoms and 19% died. Gastroenteritis was the most frequent cause of hypernatremia. All had clear evidence of dehydration. Patients have varying degrees of impaired consciousness and hyperpyrexia. Approximately one-third experience generalized convulsions and spasticity. Focal neurologic abnormalities, notably hemiparesis, are seen in approximately 10% of patients. Finberg found subdural hematomas in many of his hypernatremic infants (216). In some, neurologic symptoms, notably seizures, do not appear until 24 to 48 hours after the start of fluid therapy. These symptoms have been ascribed to cerebral edema and a lowered convulsive threshold developing with rehydration of the brain (213).
Hypernatremia may develop in diabetes insipidus if patients are unconscious or do not have access to water. It is seen in patients with defective thirst mechanism, which is usually associated with other signs of hypothalamic dysfunction. This may be a complication of surgery for suprasellar tumors such as craniopharyngioma (217). Hypernatremic patients are often severely ill and may have intracranial hemorrhage (218), leading to suspicion of child abuse (219). Rhabdomyolysis and pontine and extrapontine myelinolysis can complicated severe hypernatremia (220,221).
Potassium
Hypokalemia
Brain extracellular potassium concentration has major effects on cerebral excitability, but cerebral disturbances are extremely rare in patients with hypo- or hyperkalemia. Hypokalemia is a common metabolic disturbance but rarely causes or contributes to confusion and coma (224). Potassium depletion from any cause may produce muscle weakness. In severe cases, this progresses to quadriplegia and respiratory failure resembling Guillain-Barré syndrome (225). The effects of hypo- and hyperkalemia on muscle function and the periodic paralyses are covered in Chapter 16.
Hyperkalemia
Hyperkalemia is an often seen laboratory artifact due to hemolysis of red blood cells. True hyperkalemia is common in patients with renal or adrenal failure. Marked hyperkalemia can cause severe cardiac manifestations and weakness similar to that associated with hypokalemia. The latter can involve facial and pharyngeal muscles.
Chloride
Hypochloremia
A syndrome marked by anorexia, lethargy, failure to thrive, muscular weakness, and hypokalemic metabolic alkalosis was seen in infants who ingested a chloride-deficient formula for the preceding 1 or more months (226,227). Serum chloride as low as 61 mEq/L and arterial pH values as high as 7.74 were recorded (226). Usually, urinary chlorides were completely absent. Impaired growth of head circumference was documented in the majority of cases. Rehydration and chloride supplementation reversed all symptoms and resulted in a marked acceleration of motor milestones and in complete or partial recovery of the decelerated skull growth. Developmental testing in some of these children at 9 to 10 years of age indicated that children who had received this formula had significantly lower scores on the Wechsler Intelligence Scale for
Children (WISC) and significantly higher risks for receptive and expressive language disorders (228).
Children (WISC) and significantly higher risks for receptive and expressive language disorders (228).
Kaleita and coworkers have recognized a clinical picture of an expressive language delay, coupled with visuomotor deficits and an attention deficit disorder that often assumes the overfocused pattern (see Chapter 18). When the defect is more severe, the language and visuomotor problems can expand to assume a picture of generalized mental retardation, and the attention disorder can exhibit autistic features (229). A similar condition has been seen in nursing infants whose mothers’ milk was for unknown reasons deficient in chloride (230).
Familial chloride diarrhea, a rare hereditary disorder described in 1945, requires lifetime intake of huge amounts of chloride and responds to treatment with proton pump inhibitors (231).
Calcium
Calcium is the major extracellular divalent cation. Both high and low serum calcium levels are associated with neurologic symptoms. Total calcium in serum is found in three forms: protein bound (therefore nondiffusible, 30% to 55% of total); chelated (i.e., diffusible but nonionized, 15% of total); and ionized (remaining percentage). Generally, the appearance of neurologic symptoms correlates well with levels of ionized calcium of 2.5 mg/dL or less. The concentration of CSF calcium is normally approximately one-half that of serum calcium and represents the result of a secretory process, rather than the movement of diffusible and ionized calcium from the serum. Changes in the CSF concentration are relatively small, although large alterations in serum calcium values overcome homeostatic mechanisms.
TABLE 17.11 Conditions Producing Hypocalcemia, Hypomagnesemia, and Neurologic Symptoms | ||||||||||||||||||||||||||||||
---|---|---|---|---|---|---|---|---|---|---|---|---|---|---|---|---|---|---|---|---|---|---|---|---|---|---|---|---|---|---|
|
Hypocalcemia
The clinical picture of hypocalcemia and its causes varies with the age of the affected child. Some of the syndromes that produce hypocalcemia are outlined in Table 17.11 (232,233,234,235,236,237,238,239,240).
In the last century, hypocalcemia was one of the more common causes of seizures during the neonatal period, and it is still encountered in premature infants or stressed term infants, typically in the first days of postnatal life (232,241). The condition can be defined by a level of serum calcium below 7 mg/dL or of ionized calcium below 3.5 mg/dL. Two forms of neonatal hypocalcemia are encountered. One occurs during the first 2 days of life in premature and critically ill term infants. It also is seen in infants who have suffered perinatal asphyxia and in infants of mothers with insulin-dependent diabetes. As many as 50% of very-low-birth-weight infants have serum calcium levels below 7 mg/dL (242). The exact mechanism of this form of hypocalcemia is still obscure. Impaired vitamin D
metabolism has been excluded as a pathogenetic factor. Increased levels of calcitonin have been suggested as an etiologic factor in the hypocalcemia of prematurity, but not for that seen in infants of diabetic mothers (232,243). Less often, maternal hyperparathyroidism, congenital absence of the parathyroid glands, or disturbed renal function induce neonatal hypocalcemia (see Table 17.11).
metabolism has been excluded as a pathogenetic factor. Increased levels of calcitonin have been suggested as an etiologic factor in the hypocalcemia of prematurity, but not for that seen in infants of diabetic mothers (232,243). Less often, maternal hyperparathyroidism, congenital absence of the parathyroid glands, or disturbed renal function induce neonatal hypocalcemia (see Table 17.11).
The second form of neonatal hypocalcemia is the classic neonatal tetany (late hypocalcemia), whose mechanism was first elucidated by Bakwin in 1937 (244). It occurs between the fifth and tenth days of life and results in part from intake of cow’s milk, which induces an increased phosphate load. In this form of hypocalcemia, hyperphosphatemia and hypomagnesemia are commonly present. Additionally, low circulating parathyroid hormone levels are seen. With the widespread use of low-phosphate milk formulas, this condition has virtually disappeared. In the series of Lynch and Rust, congenital heart disease was seen in 47% of infants with hypocalcemic seizures and prematurity in 13%. Maternal hyperparathyroidism, idiopathic hypoparathyroidism, and DiGeorge syndrome were other causes. In 20% of infants, there was no obvious cause for the hypocalcemic seizures (245). In my experience, when hypocalcemia is found in a neonate with seizures, it is far more likely an association rather than its cause.
Neonatal hypomagnesemia has been recorded in connection with hypocalcemia resulting from maternal hyperparathyroidism (246). It also can be the result of a selective malabsorption of magnesium (243) (see Table 17.11).
Hypocalcemic seizures can be focal, multifocal, or generalized. In the series of Lynch and Rust, multifocal clonic seizures were the most common. True tonic seizures or tonic-clonic (grand mal) attacks are unusual, and the latter seizure type was not encountered by those researchers (245). In the interictal period, infants generally are alert, and seizures without apparent loss of consciousness are not uncommon (233). An increased extensor tone is relatively common, as are increased deep tendon reflexes and ankle clonus. Jitters were encountered in 35% of hypocalcemic infants in the 1971 series of Cockburn and coworkers (233) and in 27% of neonates in the series of Lynch and Rust (245). Patients looked relatively well between seizures, and most had good outcomes. In contrast to neonates suffering from seizures owing to nonmetabolic causes, persistent focal neurologic deficits are not observed. The classic signs of tetany seen in the older child are usually absent. Thus, carpopedal spasm was rare, and stridor owing to laryngospasm and Chvostek’s sign (a brief contraction of the facial muscles elicited by tapping the face over the seventh nerve) were not noted in any of the hypocalcemic infants reported by Keen (247).
The EEG is frequently abnormal and can demonstrate electroencephalographic seizures (245).
The treatment of seizures caused by neonatal tetany consists primarily in the administration of calcium salts (see the section on neonatal seizures in Chapter 15). The long-term outlook for infants who have experienced seizures owing to late hypocalcemia is generally good, and in the absence of subsequent neurologic insults, the majority develop normally (233,245,247). Calcium deposition in necrotic areas of brains in stressed neonates has been related to the transient elevations of ionic calcium after parenteral administration of calcium gluconate (248).
In older infants and in children, neurologic symptoms of hypocalcemia include tetany and seizures. Tetany is characterized by episodes of muscular spasms and paresthesias mainly involving the distal portion of the peripheral nerves. Episodes appear abruptly and are precipitated by hyperventilation or ischemia. No alteration of consciousness occurs. Carpopedal spasm and laryngospasm are the two most frequent examples of tonic muscular spasms. Chvostek’s sign is not diagnostic of tetany because it is seen in healthy infants and children. Seizures can occur in the absence of tetany and are occasionally focal. Headaches and extrapyramidal signs are less common and are confined to older children or adults with hypoparathyroidism (249). In this condition, CT scans can show symmetric bilateral punctate calcifications of the basal ganglia, although only 50% show an association between this finding and the occurrence of extrapyramidal signs (249). Other manifestations of hypocalcemia include laryngeal stridor (237) as well as a psychosis that responds poorly to antipsychotic drugs until calcium and magnesium have been normalized (250).
Pseudohypoparathyroidism is characterized by obesity, moon-shaped facies, mental retardation, cataracts, short and stumpy digits, enamel defects, and impaired taste and olfaction. Calcifications of the basal ganglia are seen in approximately one-third of instances. The condition is seen more commonly in females and is caused by an inability of renal tubules to respond to parathormone (251).
In neonates undergoing gastrostomy for various reasons, vitamin D malabsorption can lead to hypocalcemic seizures. This condition is treated by parenteral administration of vitamin D (252). Tetanic seizures also can result from sodium phosphate enemas (253). I have seen nonfocal seizures in the first year of life in exclusively breast-fed infants whose mothers took no milk or dairy products (254). Nutritional rickets is an occasional cause of fractures and seizures in an infant, which at times leads to an incorrect diagnosis of child abuse (255).
Hypercalcemia
Hypercalcemia may be a result of hyperparathyroidism, which is rare in children, from idiopathic hypercalcemia of infancy, Williams syndrome (256), or several rare inborn errors of metabolism (257,258). As in adults, hypercalcemia may accompany malignant disease, including leukemia (259), and immobilization in patients with end-stage renal disease (260). Hypercalcemia may be the
first sign of childhood leukemia (261). Confusion, movement disorders, and coma are seen with hypercalcemia, as with many other electrolyte disturbances. Seizures are less frequent than in hypocalcemia; they have been associated with a reversible syndrome of confusion, blindness, and seizures (262).
first sign of childhood leukemia (261). Confusion, movement disorders, and coma are seen with hypercalcemia, as with many other electrolyte disturbances. Seizures are less frequent than in hypocalcemia; they have been associated with a reversible syndrome of confusion, blindness, and seizures (262).
TABLE 17.12 Hereditary Hypomagnesemic Syndromes | ||||||||||||||||||||||||||||||
---|---|---|---|---|---|---|---|---|---|---|---|---|---|---|---|---|---|---|---|---|---|---|---|---|---|---|---|---|---|---|
|
Magnesium
Hypomagnesemia
Hypomagnesemia may be seen alone or together with hypocalcemia. Congenital hypomagnesemia is a classical cause of recurrent tetany and seizures beginning in the first weeks of life. Serum calcium and magnesium are both low, but only magnesium treatment is effective (240,263,264). The various rare hereditary syndromes associated with hypomagnesemia are listed in Table 17.12. Seizures due to renal magnesium wasting can present any time during the first year of life, although they are most common in the first week of life.
Magnesium depletion is common during cisplatin treatment and often is accompanied by significant potassium depletion (265). Cisplatin treatment may be followed by a permanent renal tubular defect with magnesium wasting, which becomes evident by convulsions or episodic encephalopathy (266). Various GI and hepatic diseases, severe malnutrition, and almost any primary disorder of calcium metabolism can cause hypomagnesemia.
Hypermagnesemia
Magnesium was once used as an anesthetic agent (267). Marked hypermagnesemia reduces transmitter release and causes muscle weakness, but not loss of consciousness (268). Magnesium is well established in the treatment of eclampsia of pregnancy (269) and also can be given intravenously for treatment of bronchospasm (270) and refractory convulsions (271). Hypermagnesemia is generally due to excessive magnesium intake that is often combined with renal failure. It has been seen in rhabdomyolysis and acute diabetic ketoacidosis. Hypermagnesemia aggravates myasthenia gravis and potentiates the effects of neuromuscular blocking agents. Calcium gluconate should be given intravenously for cardiac or respiratory manifestations of hypermagnesemia. Neonates are more sensitive to the neuromuscular blocking effects of magnesium than older children.
Phosphate
Phosphate depletion and hypophosphatemia has been associated with a rare and poorly defined encephalopathy (272,273,274). This is usually a complication of total parenteral nutrition (273,274). Patients may show tremor, confusion, agitation, ophthalmoplegia, and coma, and some are areflexic. Hypophosphatemia appears to contribute to central pontine myelinolysis in a few patients with Wernicke’s encephalopathy, including patients with hyperemesis gravidarum as the primary problem (275). Hypophosphatemia and encephalopathy have been seen in anorexic patients receiving hyperalimentation.
NEUROLOGICAL COMPLICATIONS OF GASTROINTESTINAL DISORDERS
Gastroenteritis
Gastroenteritis is associated with clusters of seizures, even in the absence of fever. The first reports of this association came from Asia (276), but I have seen cases every year since 1998 that were not limited to children of Asian background (277). Typically, a previously normal child less than
five years old begins to have GI symptoms, has a cluster of seizures with lethargy and perhaps ataxia, and recovers completely in 48 hours or so. EEG during the seizure cluster is normal or a bit slow. Encephalopathy often accompanies the seizures (278). Rotavirus is the cause in some cases (279). Rotavirus also may produce an encephalopathy with or without CSF pleocytosis. In the experience of Goldwater and coworkers, the presence of CSF pleocytosis is associated with a better outcome (280). Rotavirus also has been associated with the syndrome of hemorrhagic shock and encephalopathy (281).
five years old begins to have GI symptoms, has a cluster of seizures with lethargy and perhaps ataxia, and recovers completely in 48 hours or so. EEG during the seizure cluster is normal or a bit slow. Encephalopathy often accompanies the seizures (278). Rotavirus is the cause in some cases (279). Rotavirus also may produce an encephalopathy with or without CSF pleocytosis. In the experience of Goldwater and coworkers, the presence of CSF pleocytosis is associated with a better outcome (280). Rotavirus also has been associated with the syndrome of hemorrhagic shock and encephalopathy (281).
Celiac Disease
Wheat, rye, and barley proteins induce celiac disease, an autoimmune type of gastrointestinal disorder, in genetically susceptible persons. Subjects with celiac disease can present with cerebellar ataxia, less often with progressive myoclonic ataxia, myelopathy, or cerebral, brainstem and peripheral nerve involvement (282,283). In many cases, there is no overt malabsorption or growth problem. Epilepsy, which is frequently associated with occipital lobe calcifications, also is encountered—even in patients who are not malnourished (284)—as is an inflammatory white matter disease resembling multiple sclerosis (285). Although neurologic complications of celiac disease are generally restricted to adult subjects, children with celiac disease are at greater risk for developing learning disorders, ADHD, and headaches than control subjects (286). Patients develop various antibodies, including antigliadin, antiendomysial, and antitissue transglutaminase antibodies (anti-tTG), of which anti-tTG may be the most specific for the condition (287).
Vitamin E Deficiency States
The recognition that vitamin E deficiency is associated with a number of neurologic manifestations points to the important role played by vitamin E in normal neurologic function. Generally, vitamin E deficiency induces a spinocerebellar degeneration, resembling that seen in spinocerebellar (Friedreich) ataxia. This picture is seen in a variety of conditions marked by chronic malabsorption such as occurs in chronic cholestatic liver disease (288), cystic fibrosis, short gut, blind loop, and other intestinal syndromes (289).
Children with chronic cholestatic liver disease can develop a syndrome characterized by areflexia, gait disturbance, decreased proprioception and vibratory sensation, and gaze paresis (290). Dystonia is seen less commonly (291). Nerve conduction velocities and nerve action potential amplitudes are decreased (292). In most instances, serum vitamin E levels have been low (293). In one series, 80% of children older than 5 years of age with chronic cholestasis and vitamin E deficiency developed clinically significant neurologic abnormalities, with areflexia being the earliest sign (294). A similar clinical picture is encountered in abetalipoproteinemia (295). Here, too, vitamin E levels can be strikingly reduced (see Chapter 1). However, a normal serum vitamin E level does not exclude a deficiency in the vitamin because hyperlipidemia can produce a false elevation in serum vitamin E levels. The ratio of vitamin E to total serum lipids (cholesterol, triglycerides, and phospholipids) is considered a better indicator of vitamin E deficiency. Oral vitamin E in large doses is usually satisfactory treatment, but some patients with severe problems require injectable forms of the vitamin (292,296).
A rare autosomal recessive disorder is caused by mutations in the gene for the alpha-tocopherol transfer protein. This protein incorporates alpha-tocopherol into lipoprotein particles during their assembly in liver cells. The defect results in a decrease in serum vitamin E levels (297,298). Several allelic variants have been recognized. In some, the clinical picture resembles that of spinocerebellar ataxia with peripheral neuropathy; in others, ataxia is accompanied by retinitis pigmentosa. The age of onset can be as early as the first decade, and the disease is relentlessly progressive. Vitamin E in large doses (400 to 1,200 IU) can stabilize or improve neurologic symptoms (299). However, a few patients have developed new neurologic symptoms while receiving vitamin E and maintaining satisfactory serum vitamin E levels (300). As a rule, the earlier treatment is begun the better the outcome.
The neuropathologic picture of vitamin E deficiency regardless of cause resembles that described for vitamin E deficiency in rats and monkeys. It includes a loss of large diameter myelinated sensory axons in the spinal cord and peripheral nerves, with spheroid formation. These findings are most pronounced in the posterior columns (301). The tocopherol content of biopsied peripheral nerve is reduced in vitamin E–deficient patients with peripheral neuropathy. In some cases, chemical changes precede anatomic evidence for peripheral nerve degeneration (302). Ultrastructural evidence of electron-dense accumulations in muscle fibers also has been reported (303,304).
Inflammatory Bowel Disease
Inflammatory bowel disease (Crohn disease and ulcerative colitis) is associated with an increased risk of cerebral venous thromboses, especially during times of crisis (295,296). Kao and colleagues describe four children who developed sinovenous thrombosis coinciding with flare-ups of their ulcerative colitis (305). Markers of coagulation and fibrinolysis are often abnormal in patients with inflammatory bowel disease (306). Cerebral vasculitis is associated with Crohn disease (307), and inflammatory bowel disease is associated with higher incidence of neuropathy, myelopathy, and myopathy more so than with other
illnesses (308). Not all cases can be explained by intestinal malabsorption and an associated vitamin E deficiency. Prolonged use of metronizadole (Flagyl) for the treatment of the condition can result in a clinical or subclinical sensory polyneuropathy or a combined sensorimotor polyneuropathy.
illnesses (308). Not all cases can be explained by intestinal malabsorption and an associated vitamin E deficiency. Prolonged use of metronizadole (Flagyl) for the treatment of the condition can result in a clinical or subclinical sensory polyneuropathy or a combined sensorimotor polyneuropathy.
White matter lesions similar to those of multiple sclerosis have been seen in Crohn disease patients who were treated with the tumor necrosis factor antagonists etanercept and infliximab (309).
The association of ileal lymphoid nodular hyperplasia (ILNH) with regressive autism is covered in Chapter 18.
Whipple Disease
Whipple disease is an unusual systemic illness caused by the culture-resistant organism Tropheryma whipplei. Although usually a disease of middle-aged men, the condition has been encountered in children; it causes CNS symptoms with or without obvious gastrointestinal disease (310,311). These include cognitive changes, supranuclear gaze palsy, altered consciousness, oculomasticatory and oculofacial skeletal myorhythmia, myoclonus, ataxia, and cranial nerve abnormalities (311). Some patients have CSF pleocytosis. Diagnosis usually requires brain or intestinal biopsy (312). The treatment of Whipple disease is beyond the scope of this text.
Abdominal Compartment Syndromes
Changes in intra-abdominal pressure can influence intracranial pressure, primarily through effects on cerebral venous pressure. Citerio and coworkers showed that placing weights on the abdomen of patients with head trauma produced measurable increases in intracranial pressure (313). Intra-abdominal pressure can be significant with ascites and other abdominal fluid collections (314) and may contribute to the evolution of pseudotumor cerebri in very obese patients (315).
Pancreatic Encephalopathy
Generalized encephalopathy complicates acute pancreatitis in up to one-third of cases (316,317). Features include confusion, depression of consciousness, and occasionally asymmetrical weakness. There can be slowing of the EEG. White matter lesions are sometimes evident on MRI studies (316). If the pancreatitis subsides, neurologic recovery is likely.
Intussusception
Intussusception is relatively common in early childhood, but its diagnosis can be difficult. The classical triad of abdominal pain, currant jelly stools, and palpable abdominal mass is seen in less than one-half of patients. Whereas lethargy in gastrointestinal disorders is unexpected, approximately one-half of patients present with the symptom. Lethargy is common in volvulus as well and is seen occasionally in bowel obstruction (318,319). Many have speculated that intestinal bacteria enter the circulation when the bowel is ischemic; however, blood cultures are usually negative, and the mechanism of brain dysfunction in this condition is unknown. In a few cases, lethargy can be so profound as to prompt lumbar puncture, neurologic consultation, and brain imaging studies (320,321).
Hepatic Failure and Hepatic Encephalopathy
Liver damage by acute or chronic disease initiates a characteristic set of neuropsychiatric symptoms termed hepatic encephalopathy (HE), a condition that was first delineated in 1952 by Adams and Foley (322). It is variable in severity and multifactorial in etiology (323,324).
Pathology and Pathogenesis
In acute liver failure, the morphologic changes in the brain are dominated by astrocytic alterations, notably astrocytic swelling and cytotoxic brain edema (325). With progression of brain edema, intracranial pressure increases and ultimately results in cerebral herniation. In chronic liver failure, the principal microscopic abnormalities include enlargement and an increased number of protoplasmic astrocytes. These cells (Alzheimer II cells) are astrocytes with an enlarged, pale nucleus and a marked diminution in glial fibrillary acidic protein. They are found throughout the cerebral cortex, basal ganglia, brainstem nuclei, and the Purkinje layer of the cerebellum (322,324). They also are seen in human immunodeficiency virus (HIV) encephalopathy (see Chapter 7). Neuronal changes are generally not seen. Less often, central pontine myelinolysis has been noted in children with hepatic failure (326).
According to current consensus, HE is multifactorial and in part represents a failure of glioneural communication and cooperation (327,328,329). The two most important factors in its pathogenesis are increased plasma and brain concentrations of ammonia. The brain:blood ratio of ammonia concentrations is markedly elevated, probably a consequence of a disrupted blood–brain barrier (324). In the brain, ammonia is converted to glutamine, which cycles from astrocytes to neurons, where it is further converted to glutamate. After release of glutamate into the synaptic cleft, its reuptake occurs in astrocytes. It has been postulated that the increased synthesis of glutamine depletes available amounts of α-ketoglutarate and reduces the concentration of high-energy phosphates, thus slowing the reactions in the Krebs tricarboxylic acid cycle. Decreased oxygen consumption and glucose metabolism, however, are secondary to HE rather than causative (328).
Although in the past enhanced GABAergic neurotransmission was cited as a cause for hepatic encephalopathy, the evidence for this effect is far from convincing. In a vast majority of HE models, there are no alterations of GABA content in the brain tissue and/or extracellular space, and most of the substances in brain and other tissue with GABAergic properties can be traced to the ingestion of benzodiazepines (324).
TABLE 17.13 Stages, Signs, and Symptoms of Hepatic Encephalopathy | |||||||||||||||
---|---|---|---|---|---|---|---|---|---|---|---|---|---|---|---|
|
HE is associated with multiple secondary biochemical abnormalities. MRI studies have shown increased signal in the globus pallidus in T1-weighted images, and an increased concentration of brain manganese is believed responsible for these findings. Brain copper concentrations also are increased, and as is the case in Wilson disease, the accumulation of the toxic metals can alter astrocyte function and morphology (324).
Evidence for the synergistic role of other neurotoxins such as mercaptans, short-chain fatty acids, and phenols as well as the generation of false neurotransmitters such as octopamine is currently less strong (328). Additionally, liver failure induces profound multisystem disturbances, which, in turn, can further impair neurologic function (330). Of significance are gut-derived bacteria and their toxic products, which are known to injure the liver and cause systemic illness (331,332,333). Serum levels of proinflammatory cytokines are increased in hepatic encephalopathy, and the severity of encephalopathy has been correlated with serum levels of tumor necrosis factor α (334).
Clinical Manifestations
HE can occur in two forms: acutely, as in fulminant hepatic failure, and as a chronic, progressive encephalopathy. In children, acute hepatic failure is primarily responsible for clinically important HE (335). The most common predisposing causes are acute infectious hepatitis; Wilson disease (336); the ingestion of various drugs such as valproic acid, acetaminophen, isoniazid, or halothane; and toxins, notably mushroom poisoning (337). In infancy, galactosemia, fructosemia, or tyrosinemia can present as fulminant hepatic failure (see Chapter 1). In the past, Reye syndrome and hemorrhagic shock syndrome presented with fulminant liver failure (see Chapter 7).
The onset of the encephalopathy usually coincides with a deterioration of the general clinical condition. The principal signs and symptoms of hepatic coma are related to disorders of consciousness. Neurologic symptoms in hepatic failure generally can be divided into six groups: those due to hypoglycemia; sepsis; intracranial bleeding resulting from coagulopathies; renal failure; electrolyte disturbances, notably hyponatremia, hypokalemia, and hypocalcemia; and cerebral edema. The various stages of HE are outlined in Table 17.13.
It is of utmost importance that the first signs of encephalopathy are recognized, as the progression from stages I to IV can be exceedingly rapid. Because the first evidence of encephalopathy may be outbursts of violent agitation or uncharacteristic behavior, the early stage of HE is frequently misdiagnosed. Hyperventilation can develop during stages II and III and can lead to alkalosis, low serum pCO2, and a further deterioration of mental status. A fine tremor, and more characteristically coarse flapping movements, termed asterixis, can be present in stages I and II, respectively, whereas decorticate and decerebrate postural responses accompany stage IV of HE. Choreiform movements, a fluctuating rigidity of the limbs, parkinism, dystonia, and periods of noisy delirium are particularly frequent asian indian (338,339).
Poddar and colleagues reported 67 Asian-Indian children with fulminant hepatic failure treated without transplantation, 63 of whom were thought to have viral hepatitis (340). All children with stage I or II encephalopathy survived. Seventeen of 36 children with stage III or IV encephalopathy died. Ascites, peritonitis, and chemical evidence of severe hepatocellular dysfunction all increased the risk of death. Cerebral edema is a prominent part of the clinical picture of acute HE and is the principal cause of death, with brainstem herniation found in up to 80% of patients dying in fulminant hepatic failure (341).
Although severe liver disease is a prerequisite for the appearance of HE, ascites, jaundice, edema, or hepatomegaly do not invariably accompany the neurologic involvement. In fact, frequently, as irreversible liver failure supervenes, previously elevated serum transaminase levels decrease rapidly, the coagulopathy worsens, the initially enlarged liver shrinks, and the total bilirubin climbs while the conjugated portion decreases.
In a majority of patients, the EEG shows paroxysmal and diffuse bursts of high-voltage slow-wave activity, a pattern that is not specific for HE but is highly indicative of one of the metabolic encephalopathies. Triphasic waves, characteristic for HE, are common in adults but rare in children. Clinical or EEG evidence of seizures is associated with a poor outcome (342).
Treatment and Prognosis
The advent of successful liver transplantation, which offers a success rate of between 55% and 89%, has revolutionized the management, treatment, and prognosis of children with liver failure and HE (343,344). Liver transplantation is the definitive treatment in patients with acute or chronic hepatic failure, and in several major centers, including our own, results have been encouraging both in terms of survival rate and post-transplant complications. The decision of which patients to transplant and when surgery is to be done is beyond the scope of this text. Suffice it to say that the mortality of patients in stage IV HE is 63% to 80%. In particular, patients who have developed cerebral edema fare badly and are not suitable candidates for liver transplant (344). In the experience of the Children’s Hospital of Pittsburgh, 70% of children who developed cerebral edema as demonstrated by computed tomography (CT) scan died, and 15% were left with severe to profound neurologic deficits. The remainder were left with moderate deficits that prevented them from an independent lifestyle (344).
The child with HE requires meticulous medical management until either the liver resumes adequate function or a replacement organ is found. Management of the precipitating event, dietary protein restriction, avoidance of constipation, and alteration of the intestinal flora are the major aspects of the therapeutic regimen (345). The therapeutic value of flumazenil, a benzodiazepine antagonist, appears to be minimal in the pediatric population (346). Response to flumazenil is transient, and the medication may precipitate an anaphylactic reaction.
Most commonly, the neurologist becomes involved in the treatment of a child with HE who has developed cerebral edema. Because cerebral edema mainly occurs on a cytotoxic basis, corticosteroids are not recommended for its treatment and were found to be ineffective in controlled trials. The best course of management is by fluid restriction and assisted hyperventilation. Hyperventilation is widely used and seems helpful early in the course of the illness. Cerebral blood flow tends to increase as encephalopathy develops if ventilation is not controlled, and this relative hyperemia contributes to intracranial hypertension (347). Late in the course of hepatic failure, cerebral blood flow tends to decrease, and hyperventilation at this stage can be harmful (348). Mannitol and related osmotic diuretics are helpful, but prolonged use of mannitol can cause hyperosmolality and aggravate renal problems (349,350). Minimizing stimulation (lights, sound, endotracheal suctioning) avoids sharp increases in intracranial pressure that are liable to become sustained and recalcitrant to therapeutic measures. Short-acting narcotics (fentanyl) can be administered to further blunt intracranial pressure increase from stimulation. Hypothermia and barbiturate coma have been advocated but should only be used if intracranial pressure is monitored.
Extradural or subdural monitoring devices are increasingly used for children in stage III or IV (350). These allow management of intracranial pressure and permit documentation of cerebral hypoperfusion and the rapid fluctuations of intracranial pressure encountered during the transplant procedure (351). Placement requires an experienced neurosurgeon and often necessitates simultaneous administration of fresh frozen plasma. In the experience of Blei and coworkers, a potentially fatal hemorrhage was the most common complication of cerebral pressure monitoring in fulminant hepatic failure (352).
This is not the place to discuss the complexities of artificial liver support (353) and the details of managing severe hepatic failure. For a comprehensive discussion of the therapy of hepatic failure, the interested reader is referred to reviews by Jalan (354), Sherlock (349), and DeVictor and coworkers (350).
Suffice it to say that hepatic transplantation is no solution unless intracranial pressure is controlled. Sustained increases in intracranial pressure resulting in diminished cerebral perfusion pressure (less than 40 torr for more than 2 hours) are generally accepted as a contraindication to liver transplantation (355). In addition, the cause of hepatic failure and a variety of other prognostic indicators must be considered (356). In particular, the longer the interval between the onset of jaundice and the development of HE, the worse the outcome (357). As a rule, somatosensory-evoked potentials are superior to EEG in terms of prognosis, and a lack of a thalamocortical potential presages a poor outcome (358).
NEUROLOGIC COMPLICATIONS OF LIVER TRANSPLANTATION
Transplantation has revolutionized the management of severe hepatic failure; however, neurologic complications of liver transplantation occur in 30% to 60% of patients (359).
As a rule, neurologic complications occur in the first months following transplantation (360). They can be categorized into problems related to the underlying disease, problems related to the transplant procedure, side effects of immunosuppressive drugs, and neurologic complications arising from immunosuppression (361).
As a rule, neurologic complications occur in the first months following transplantation (360). They can be categorized into problems related to the underlying disease, problems related to the transplant procedure, side effects of immunosuppressive drugs, and neurologic complications arising from immunosuppression (361).
The complications arising from the transplant procedure are related to the major fluid and electrolyte shifts that can occur during surgery when the diseased liver is removed and reperfusion of the new liver may produce a return of intracranial hypertension (362). Intracranial hemorrhage secondary to coagulopathy and severe ischemic injury secondary to hypoperfusion are uncommon but devastating consequences. Patients with fulminant hepatic failure can continue to experience encephalopathy and life-threatening cerebral edema for several days after the transplant. Intraoperative intracranial pressure can increase secondary to the stress of surgery, supine operating position, or fluid shifts.
Initial encephalopathy can occur in up to 60% of transplants (363). Seizures are less common (363); in a few instances, rapid shifts of sodium or other electrolytes during the perioperative period are associated with central pontine myelinolysis (364,365). Intracranial hemorrhage and ischemic strokes are most often encountered in patients with major infection (366,367).
In the pediatric age group, infectious complications resulting from immunosuppression are the most common, with the risk for CNS infections being the greatest between 1 and 6 months following transplantation (368,369). These may take the form of acute meningitis, subacute chronic meningitis, a meningoencephalitis, or a brain abscess. Conti and Rubin provide a timetable for the occurrence of infections in the transplant patient (368). Opportunistic infections of the CNS are rare during the first month after transplantation. The organisms responsible are different from those found in immunocompetent children. Four organisms—Listeria monocytogenes, Aspergillus fumigatus, herpesviruses, and Cryptococcus neoformans—account for most cases, although there is geographic variation (370). At 6 months post-transplant, patients are at risk for cryptococcal meningitis and cytomegalovirus. The most common organism to cause acute meningitis in the immunocompromised child is Listeria monocytogenes. Cryptococcus, Listeria, and Mycobacterium tuberculosis can be responsible for subacute infections. Brain abscesses are much more often due to fungal than bacterial infection in this special population (369).
Neurologic symptoms also can result from the use of immunosuppressive agents. Cyclosporine induces neurologic symptoms in some 10% to 25% of patients (370). The most common neurologic symptom is tremor. This can be caused by sympathetic activation, a leukoencephalopathy, or can be a part of generalized cerebellar dysfunction (371). Less often, one can observe seizures. In some patients, these appear to be related to metabolic derangements, notably hypomagnesemia. Seizures are more likely to occur with the intravenous form of cyclosporine (Sandimmune) than with the oral form of the drug. In such cases, the neurologic consultant is often asked whether to start anticonvulsant therapy as well as which anticonvulsant is most appropriate. By inducing the hepatic P450 system, most anticonvulsants interfere with the metabolism of immunosuppressive agents, thus increasing their required dosage. Benzodiazepines, gabapentin, and valproate are the drugs of choice in that they tend to have a lesser effect on cyclosporine metabolism (360).
A relatively frequent condition termed reversible posterior encephalopathy (RPE) has been seen in children receiving immunosuppressants such as cyclosporine or tacrolimus (372). This complication is more common when high doses are used, but there is no specific blood level that separates affected and unaffected patients receiving these drugs. Hypertension is almost invariably present, but it may be surprisingly mild. Its pathophysiology is similar to that of hypertensive encephalopathy in that the condition results when systemic blood pressure exceeds the autoregulatory capacity of the cerebral vasculature, with consequent breakdown of the blood–brain barrier and transudation of fluid into the brain. It is believed that the relative lack of sympathetic innervation of the posterior circulation may predispose the parietal occipital region to vasodilatation and breakdown of the blood–brain barrier. The condition is reversible upon lowering the blood pressure or discontinuation of immune suppressants. This entity presents with severe headache, depression of consciousness, seizures, confusion, or cortical visual deficits (373). It is rarely associated with papilledema or retinal hemorrhages. Many patients have seizures during the acute phase, but few have epileptiform discharges in their EEGs, and the majority can be withdrawn from anticonvulsant medication after 4 to 6 months. The CSF is generally normal (374). The neuroimaging picture is characteristic in that it demonstrates a symmetric bilateral subcortical/cortical hyperintensity in T2-weighted images typical of vasogenic edema (375) (Fig. 17.1). RPE is seen not only in patients on immunosuppressants, but also in a wide variety of other disorders that include acute glomerulonephritis with relatively normal creatinine, eclampsia of pregnancy, and uncontrolled hypertension. It also is encountered in patients receiving cancer chemotherapy (376,377,378). RPE is generally reversed after discontinuation or reduction of the immune suppressant. It has been linked to low serum cholesterol levels in that hypocholesterolemia upregulates the low-density lipoprotein receptor, which increases intracellular transport of cyclosporine (379). Although most patients do well, some are left with permanent neurologic deficits (380,381).
Patients taking immunosuppressant drugs should generally not receive enzyme-inducing antiepileptic drugs because they decrease the efficacy of the immunosuppressant agents. Benzodiazepines, valproate, gabapentin, and levetiracetam have been used successfully in transplant patients (382,383), but only the first two drugs are currently available in injectable form.
Other serious complications of immunosuppressant therapy include cerebellar symptoms, mental confusion, polyneuropathy, a motor spinal cord syndrome, and thromboembolic phenomena. A dose-dependent myopathy has been encountered some 5 to 25 months after initiation of cyclosporine therapy (384). In a small proportion of patients, cyclosporine induces a hemolytic uremic syndrome or thrombotic thrombocytopenic purpura with ensuing neurologic symptoms (385).
Tacrolimus is a newer immunosuppressant that is used increasingly in children. The spectrum and incidence of neurotoxicity is similar to that of cyclosporine. A severe postural tremor and, less frequently, mutism and speech apraxia as well as seizures are the most common manifestations (386,387). Headaches also are commonly seen, especially early after transplantation, a time when immunosuppression is highest. OKT3 is a monoclonal murine IgG immunoglobulin used for short courses in severe acute organ rejection. This agent can induce a sterile CSF pleocytosis. Symptoms include fever, headache, photophobia, meningism, cerebral edema, and transient hemiparesis. They are reversed by cessation of OKT3 treatment (388,389).
At dosages required for immune suppression, corticosteroids have the potential to induce mental status changes, a steroid myopathy, cerebrovascular changes owing to hypertension, and pseudotumor cerebri, which can develop after corticosteroid withdrawal. A primary CNS lymphoma is seen in some 2% of organ transplant patients.
Parkinsonian symptoms of bradykinesia and hypokinesia, cogwheel rigidity, and resting tremor have been noted after bone marrow transplantation (390). In the series of Martinez and colleagues, the most common pathologic findings in children dying after having undergone a liver transplantation were cerebral edema; a variety of ischemic and hemorrhagic vascular lesions; and infections, mainly caused by cytomegalovirus, aspergillosis, and candidiasis (391). A liver transplant series reported from the University of California, Los Angeles, UCLA School of Medicine shows similar neuropathologic sequelae (392).
Children who are long-term survivors of liver transplantation after chronic hepatic failure are at greater risk for intellectual and neuropsychologic deficits than are children with other chronic illnesses. Whether this is an effect on cognitive functioning of the pretransplant hepatic disease or the post-transplant immune suppressant therapy remains to be established (393,394). Cognitive outcome is influenced by many factors, including the severity of liver disease before transplantation, medication effects, and various socioenvironmental factors.
NEUROLOGIC COMPLICATIONS OF RENAL DISEASE
Uremia
Pathology
The molecular basis for uremic encephalopathy remains complex and poorly understood; it is generally accepted that several toxins are responsible. Urea is the most studied of these. However, it has been known for some time that the severity of cerebral symptoms correlates poorly with levels of serum urea, and hemodialysis sometimes reverses symptoms without lowering blood urea (395). Creatinine, p-cresol, the guanidines, organic acids,
phosphates, and secondary hyperparathyroidism also are believed to contribute to the encephalopathy. Parathormone is believed to be responsible for some aspect of the various neurologic symptoms encountered in uremia, notably the peripheral neuropathy and the myopathy (396). Cerebral blood flow studies have shown a defect in oxygen use. In part, this defect might be caused by nonspecific increases in brain permeability and disordered membrane function, which could allow toxic products, possibly a variety of organic acids, to enter the brain. These acids could alter the function of the sodium-potassium ion pump. Disorders in blood and CSF electrolytes can aggravate the clinical picture, as can bouts of acute hypertensive encephalopathy (397).
phosphates, and secondary hyperparathyroidism also are believed to contribute to the encephalopathy. Parathormone is believed to be responsible for some aspect of the various neurologic symptoms encountered in uremia, notably the peripheral neuropathy and the myopathy (396). Cerebral blood flow studies have shown a defect in oxygen use. In part, this defect might be caused by nonspecific increases in brain permeability and disordered membrane function, which could allow toxic products, possibly a variety of organic acids, to enter the brain. These acids could alter the function of the sodium-potassium ion pump. Disorders in blood and CSF electrolytes can aggravate the clinical picture, as can bouts of acute hypertensive encephalopathy (397).
Clinical Manifestations
The principal neurologic symptoms of uremia are abnormalities in mental status, tremor, myoclonus, asterixis, convulsions, and muscle cramps (239,398). As a rule, the more rapidly renal failure develops, the more prominent the motor symptoms, which may include unclassifiable twitches that are bilateral but asynchronous, and seizures. The motor disorder is usually relatively symmetrical. Peripheral nerve involvement is common in patients with uremia. Most frequently, it takes the form of a polyneuropathy. This can be a symptomatic mixed motor and sensory neuropathy, or it can be subclinical, detected only by nerve conduction studies. In one report, 76% of uremic children had a significantly reduced peroneal motor nerve conduction velocity without any clinical evidence of neuropathy (399). When symptoms develop, they begin with sensory abnormalities in the lower extremities. The condition can progress slowly to total flaccid quadriplegia. Nerve biopsy can reveal primarily an axonal neuropathy, progressive axonal neuropathy with secondary demyelination, or predominantly demyelinating neuropathy (400). Less commonly, patients develop a mononeuropathy, cranial nerve palsies, and choreoathetosis. Restless legs syndrome is seen in a large proportion of uremic patients (401). Signs of hypocalcemia and hypomagnesemia are often present. On rare occasions, one can encounter a primary myopathy (402).
EEGs are generally slowed and sometimes include spike-wave discharges in patients without clinical seizures (403). Rhythmical EEG discharges without clinical seizures are more common in renal failure than in children without renal disease; many of these patients do not demonstrate paroxysmal discharges when monitored (404).
In hypertensive encephalopathy, such as occurs with acute glomerulonephritis, patients develop symptoms and signs of increased intracranial pressure, with headache, vomiting, disturbance of vision, and papilledema. Seizures and transient focal cerebral syndromes, including hemiparesis and cortical blindness, also are common.
As a rule, developmental quotients of children who develop chronic renal failure before 1 year of age are more affected than those of children who go into uremia after 3 years of age (405). In the experience of McGraw and Haka-Ikse, more than 50% of patients with chronic renal failure present since infancy had significant developmental delay (406). This is accompanied by a significant reduction in the head circumference.
Neuroimaging studies of the brain of patients with end-stage uremia reveal a high incidence of cerebral atrophy, suggesting an adverse effect of uremia on brain development (407). MRI of patients with cortical blindness demonstrates increased signals in occipital white matter and cortex on T2-weighted images. With treatment, these tend to resolve over the ensuing weeks (408). The neurologic symptoms in uremia have been reviewed by Fraser and Arieff (409) and Smogorzewski (410).
Treatment and Prognosis
Treatment of uremia involves correction of electrolyte disturbance and maintenance of normal plasma composition. These have been greatly assisted by the use of dialysis. In some instances, neurologic symptoms can become aggravated after peritoneal dialysis or hemodialysis. Some workers have suggested that urea in the brain does not equilibrate freely with urea in blood, and therefore water enters the brain along an osmotic gradient. This is generally referred to as the dialysis-dysequilibrium syndrome. Gradual changes in blood electrolytes and earlier dialysis prevent some neurologic complications. In general, motor symptoms tend to improve once blood urea levels are lowered, whereas sensory symptoms tend to remain fixed. The sensory neuropathy does, however, respond dramatically to renal transplant (411). The correction of anemia by means of recombinant erythropoietin improves intellectual function (412).
Successful renal transplantation is associated with acceleration in head growth and improved intellectual functioning. Improvement can continue for more than 1 year after the transplant (413,414). Nevertheless, prospective studies of children with moderate to severe congenital renal disease indicate that both cognitive and motor developmental delay is common. Children who develop uremia in the first year of life often have microcephaly and significant cognitive impairment even if dialyzed and later transplanted (406,415). The younger the child, the greater the risk of this complication (416). This delay in brain growth reflects, in part, a toxic effect of uremia on brain growth and maturation, and, in part, chronic malnutrition, the various metabolic disturbances, and also any antecedent brain malformation. In the series of Bock and coworkers, approximately one-half of infants with congenital
renal disease maintained normal development. In the remainder, development was delayed or deteriorated. Neither the cause for the renal disease nor its severity influenced the neurologic or cognitive status (417). Successful renal transplantation is associated with head growth and intellectual improvement (418,419). However, not all studies find better cognitive outcome following transplantation than that following dialysis (420).
renal disease maintained normal development. In the remainder, development was delayed or deteriorated. Neither the cause for the renal disease nor its severity influenced the neurologic or cognitive status (417). Successful renal transplantation is associated with head growth and intellectual improvement (418,419). However, not all studies find better cognitive outcome following transplantation than that following dialysis (420).
Treatment of convulsions in uremic patients depends on the cause of the seizures. Seizures accompanying the dysequilibrium states are usually self-limiting and often can be prevented by close supervision of dialysis. Chronic seizures can be treated with phenobarbital or phenytoin, with recognition that serum protein binding, particularly for phenytoin, is reduced in uremia. As a result, therapeutic as well as toxic effects of the drug are encountered at lower serum levels than in patients who have normal renal function. Nevertheless, anticonvulsant activity can usually be achieved with the usual doses because the free fraction of phenytoin remains unchanged (421). No modification of carbamazepine clearance or bioavailability has been observed. In renal failure, the free fraction of valproate increases two- to threefold. However, the intrinsic metabolism of the drug is reduced so that the actual clearance remains normal. The metabolism of the various benzodiazepines is unaffected. Renal clearance of levetiracetam is up to 70% reduced in severe renal impairment; clearance of the drug generally correlates with creatinine clearance. Both phenytoin and phenobarbital are known to hasten the metabolism of corticosteroids and the immunosuppressant drugs cyclosporine and FK506. This causes ineffective immunosuppression in renal transplant recipients and reduced cadaver allograft survival (422). Hence, Wassner and coworkers have suggested that anticonvulsants not be administered to patients right after transplant unless absolutely essential, and if they are given, corticosteroid dosage should be increased accordingly (422). Alternatively, a benzodiazepine can be used. The various drug interactions are considered by Cutler (423). (See Chapter 14 for a discussion of anticonvulsant therapy of patients with renal failure.)
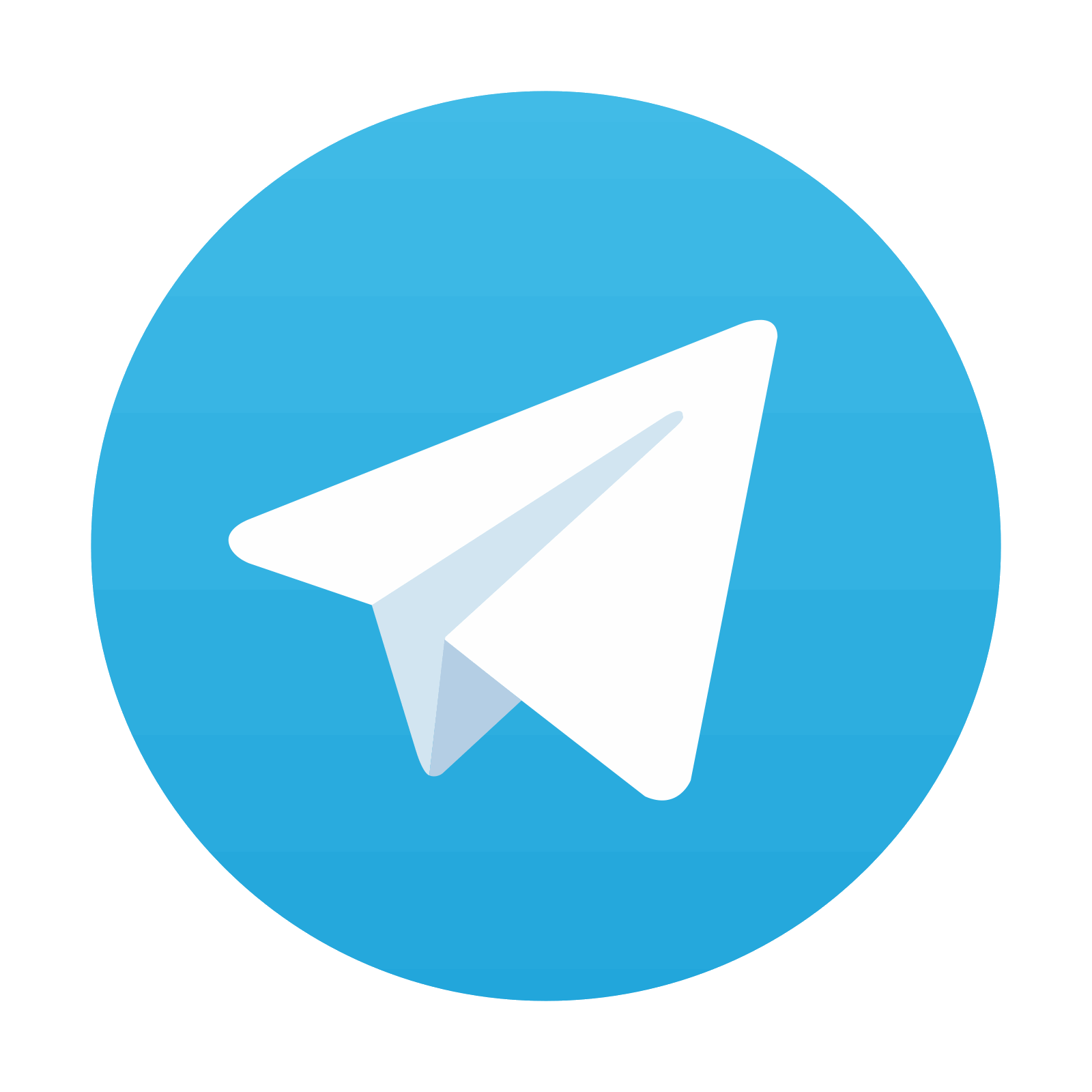
Stay updated, free articles. Join our Telegram channel
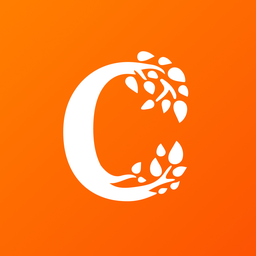
Full access? Get Clinical Tree
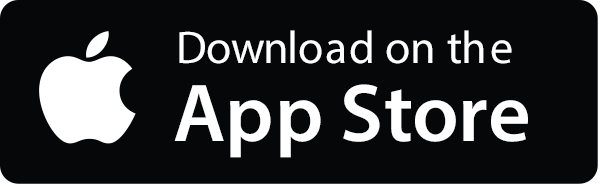
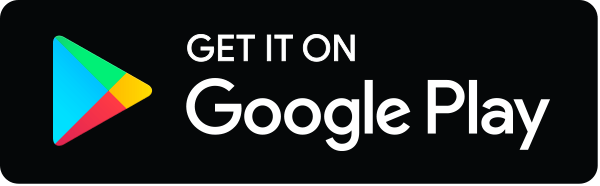
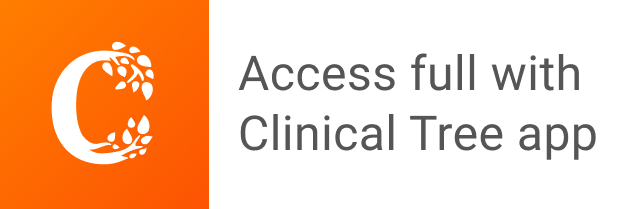