Neuromodulation of Seizures, Epileptogenesis, and Epilepsy
Helen E. Scharfman
Robert Schwarcz
Introduction
Epilepsy is a complex disease that is influenced by a large number of diverse variables. Some of these factors, such as genes that influence seizure susceptibility, are internal. Others, which alter normal brain excitability, such as traumatic brain injury, are external.
These variables can have different and distinct effects. For example, genes may influence both the development of the disorder (epileptogenesis), or the frequency and severity of seizures after epilepsy is established. Furthermore, these same factors may also influence treatment, because they can alter the efficacy of antiepileptic drugs.
A broad range of endogenous factors include a subset that can be termed “neuromodulators.” These factors are essential components of the normal central nervous system (CNS) and play an important role in the balance of excitation and inhibition in the normal brain. Here, we review neuromodulatory compounds and principles that best exemplify those that affect seizure susceptibility, epileptogenesis, and epilepsy.
In this chapter, neuromodulators are categorized into proteins and small molecules that are (a) primarily expressed in, or released from, neurons; (b) present in the extracellular space as part of the milieu that is commonly referred to as the extracellular matrix (ECM); and (c) primarily associated with astrocytes.
This review emphasizes neuromodulators that influence excitability in the hippocampus and the adjacent parahippocampal region (including the entorhinal cortex), two brain regions known to be centrally involved in limbic seizure activity. This focus does not imply that neuromodulation is most robust in those areas, but rather reflects the fact that the majority of information in the field of epilepsy research derives from these limbic regions. This includes studies in humans, in which neuromodulation has been mostly examined using surgically resected hippocampal and parahippocampal tissue from patients with intractable temporal lobe epilepsy (TLE).
In view of the complexity of the subject matter, often involving multiple receptors as well as intricate modes of regulation of expression and release for any given neuromodulator, only specific examples will be discussed in detail in each category. Furthermore, a major message of this overview is that no single neuromodulator influences excitability in a simple manner, and that cross-talk between modulators is likely to be associated with seizure activity.
Tables 1,2,3,4 provide bulleted lists of the neuromodulator categories that have been associated with pathophysiologically or therapeutically relevant aspects of seizures or epilepsy. The selection included here should be viewed merely as a snapshot of current knowledge. There can be little doubt that additional members of these families will become relevant as well, and that novel, important neuromodulators will be identified in the future.
Neuron-derived neuromodulators
Neuropeptides
Neuropeptides comprise a variety of small proteins that were originally shown to be coexpressed with classical neurotransmitters in neurons; it was assumed that this coexpression indicated a role in synaptic transmission. Accordingly, many studies focused on the mechanism(s) by which peptides might exert their effects in concert with colocalized classical transmitters such as γ-aminobutyric acid (GABA) or glutamate. It is widely recognized, however, that neuropeptides have effects independent of classical transmitters. These effects include an influence on ion channels, synaptic transmission, and hence excitability. Neuropeptides also affect growth, proliferation, vasculature, and neurogenesis, although these effects may only occur at a certain time during development or after an injurious insult.
A discussion of neuropeptides is particularly germane to epilepsy because many have robust effects on seizure threshold, seizure susceptibility, epileptogenesis, and epilepsy (i.e., the state of spontaneous, recurrent seizures). However, the concept that neuropeptides modulate seizures—and potentially epilepsy—did not arise from studying their basic, fundamental properties as cotransmitters. Instead, it was initiated by reports that showed that seizures alter the expression of neuropeptides and their receptors. This was first demonstrated in laboratory animals,80,138 although seizure-induced alterations in neuropeptides have also been found in surgically removed brain tissue from patients with intractable TLE.50
To date, it is still widely debated whether these changes in neuropeptide expression reflect a mechanism to repair the brain after seizure-induced neuronal damage or whether altered levels of neuropeptides indicate a compensatory, anticonvulsant response of the brain to prevent further seizure activity. An intriguing suggestion is that these changes are due to a pattern of genomic responses set into play to recapitulate developmental patterns.
Table 1 lists a large number of neuropeptides, organized according to the initial studies describing their expression and function throughout the body. Notably, these perspectives have evolved over time, because many of these peptides were identified in more than one brain area and found to have multiple functions.204
Figure 1 illustrates a focused view of these neuropeptides, using the dentate gyrus as an example. The dentate gyrus harbors a variety of GABAergic interneurons, which normally play an important role in preventing abnormal excitability. These neurons also contain one or more neuropeptides. In addition,
two principal cells—the abundant granule cells and the less numerous hilar “mossy cells”—use glutamate as a neurotransmitter and also express various peptides, as well as other neuromodulators. This subregion of the hippocampus plays a central role in seizure generation and propagation in animals and humans, and has been studied extensively with regard to the neuromodulatory effects of peptides in epilepsy.
two principal cells—the abundant granule cells and the less numerous hilar “mossy cells”—use glutamate as a neurotransmitter and also express various peptides, as well as other neuromodulators. This subregion of the hippocampus plays a central role in seizure generation and propagation in animals and humans, and has been studied extensively with regard to the neuromodulatory effects of peptides in epilepsy.
Table 1 Neuropeptides | |||||||||||||||||||||||||||||||||||||||||||||||||||||||||||||||||||||||||||||||||||||||||||||||||||||||||||||||||||||||||||||||||||||||||||||||||||||||||||||||||||||||||||||||||||||||||||||||||||||||||||||||||||||||||||||||||||||||||||||||||||||||||||||||||||||||||||||||||||||||||||||
---|---|---|---|---|---|---|---|---|---|---|---|---|---|---|---|---|---|---|---|---|---|---|---|---|---|---|---|---|---|---|---|---|---|---|---|---|---|---|---|---|---|---|---|---|---|---|---|---|---|---|---|---|---|---|---|---|---|---|---|---|---|---|---|---|---|---|---|---|---|---|---|---|---|---|---|---|---|---|---|---|---|---|---|---|---|---|---|---|---|---|---|---|---|---|---|---|---|---|---|---|---|---|---|---|---|---|---|---|---|---|---|---|---|---|---|---|---|---|---|---|---|---|---|---|---|---|---|---|---|---|---|---|---|---|---|---|---|---|---|---|---|---|---|---|---|---|---|---|---|---|---|---|---|---|---|---|---|---|---|---|---|---|---|---|---|---|---|---|---|---|---|---|---|---|---|---|---|---|---|---|---|---|---|---|---|---|---|---|---|---|---|---|---|---|---|---|---|---|---|---|---|---|---|---|---|---|---|---|---|---|---|---|---|---|---|---|---|---|---|---|---|---|---|---|---|---|---|---|---|---|---|---|---|---|---|---|---|---|---|---|---|---|---|---|---|---|---|---|---|---|---|---|---|---|---|---|---|---|---|---|---|---|---|---|---|---|---|---|---|---|---|---|---|---|---|---|---|---|---|---|---|---|---|---|---|
|
![]() FIGURE 1. Circuit elements and neuropeptides in the rat dentate gyrus under normal conditions. A: Major components of dentate gyrus circuitry. These include the dense layer of granule cells (GC); diverse GABAergic “interneurons” (INT), hilar mossy cells (MC), as well as precursors to granule cells (PRE) that are located in the subgranular zone. The major afferent input to the dentate gyrus is the perforant pathway, which contains the axons of neurons in the entorhinal cortex. Lateral entorhinal neurons innervate the outer molecular layer (outer) and medial entorhinal neurons innervate the medial molecular layer (medial). Mossy cells and other fibers innervate the inner molecular layer (inner). In addition, inputs to the dentate gyrus also arise from area CA3 pyramidal cells, the septum, dorsal raphe, locus coeruleus, mammillary bodies, and other areas. The main projections from the dentate gyrus are from the granule cell axons (the mossy fibers), which terminate locally on hilar processes and the layer containing the proximal dendrites of CA3 pyramidal cells (stratum lucidum; not shown). In addition, mossy cells project both ipsilaterally and contralaterally to the dentate gyrus. Some GABAergic neurons also project contralaterally. Reproduced with permission from Freund TF, Buzsaki G. Interneurons of the hippocampus. Hippocampus. 1996;6:347–470; and Scharfman HE. The role of nonprincipal cells in dentate gyrus excitability and its relevance to animal models of epilepsy and TLE. In: Delgado-Esqueta AV, Wilson W, Olsen RW, Porter RJ, editors. Basic mechanisms of the epilepsies: molecular and cellular approaches, 3rd ed. New York: Lippincott-Raven; 1999:805-820.77,179 B: Many neuropeptides are expressed in the normal dentate gyrus, and some of the most well-studied examples are shown.77 Opiates are mainly expressed in granule cells. In contrast, other neuropeptides are primarily present in interneurons. Basket cells contain both vasoactive intestinal polypeptide and cholecystokinin; hilar neurons that innervate the outer molecular layer (as well as other targets) express neuropeptide Y and/or somatostatin; vasoactive intestinal polypeptide and calretinin that define a population that innervates the inner molecular layer, as well as other interneurons; in addition, other vasoactive intestinal polypeptide-containing cells exist and may be coupled by gap junctions.90 Recent studies have indicated that substance P has widespread expression in interneurons.197 (See color insert.) |
Neuropeptide Y
Neuropeptide Y is the first example. This peptide is normally expressed in a subset of GABAergic neurons in the dentate gyrus. The axons of these cells project to numerous areas of the region and thus exert multiple effects (Fig. 2A). A primary effect of neuropeptide Y is to reduce excitatory transmission from granule cells to their targets, thereby decreasing the excitatory output of the dentate gyrus to hippocampal pyramidal cells. Thus, when synthetic neuropeptide Y is applied to slices of rodent dentate gyrus, it inhibits the excitatory output of granule cells by acting on neuropeptide Y receptors on the terminals of granule cell axons. This function is likely to be important in the control of seizure activity in the hippocampus since overexpression of neuropeptide Y is anticonvulsant227 and because decreased neuropeptide Y expression leads to increased seizure susceptibility.13
Neuropeptide Y also has other actions in the dentate gyrus. For example, it modulates calcium entry into granule cells,139 and also affects a specific potassium ion (K+) channel on GABAergic neurons in the dentate gyrus.156 The latter could influence neuronal firing and GABA release and therefore modulate the targets of GABAergic neurons.
![]() FIGURE 2. Changes in neuropeptide Y and somatostatin in the dentate gyrus after seizures. A: Normal circuitry and expression of neuropeptide Y and somatostatin in the rodent dentate gyrus. B: Alterations in neuropeptide Y and somatostatin in animal models of epilepsy are illustrated schematically. For the neuropeptide Y system, these changes include: (a) new expression in granule cells and their axons, the mossy fibers; (b) increased expression in interneurons that normally express neuropeptide Y—this effect appears to develop mostly after acute rather than chronic seizures; (c) novel expression of neuropeptide Y in some mossy cells;88 and (d) expression in newly formed granule cells, although the extent that this occurs is unclear. In addition, changes in receptors occur (not shown). For the somatostatin system, the best documented alteration is seizure-induced cell death of those interneurons that normally express this peptide. (See color insert.) |
Neuropeptide Y also facilitates the proliferation of new granule cells in the adult brain, and thus influences adult neurogenesis.88 These actions appear to be mediated by the Y1 subtype of neuropeptide Y receptors, which are situated on the proliferating cells. The source of neuropeptide Y may be the GABA/neuropeptide Y coexpressing interneurons, which have axon terminals in the subgranular zone, where the proliferating cells are located.52
Neuropeptide Y function may change after seizures, as initially suggested by studies showing that seizures affect the expression of neuropeptide Y in granule cells. Particularly after chronic seizures, the axons of the granule cells strongly express neuropeptide Y (Fig. 2B).11,129
This phenomenon may be functionally significant for two reasons: first, neuropeptide Y may exert more robust effects on the normal targets of granule cell axons; second, neuropeptide Y may have additional effects at new locations, because the axons of granule cells (mossy fibers) make new connections after chronic seizures (“mossy fiber sprouting”). Indeed, neuropeptide Y inhibits the effects of glutamate released from the sprouted axons of granule cells in epileptic rodents.218 These data support the concept that neuropeptide Y is “anticonvulsant” and its upregulation after seizures is “compensatory” in nature. Interestingly, acute seizures, as opposed to chronic seizures, appear to have different effects on neuropeptide Y expression. Thus, a brief seizure results in a preferential, transient increase in neuropeptide Y expression in GABAergic neurons,200 but does not cause robust changes in neuropeptide Y expression in granule cells. The functional role of the increase in neuropeptide Y within GABAergic neurons is still not clear, but it could be a way to inhibit glutamate release, which could prevent another seizure. It is important to add that this transient increase of neuropeptide Y in GABAergic neurons may be underestimated, because seizures may injure neuropeptide Y–containing neurons.29,49,212
Additional complications arise from the fact that some, but not all, neuropeptide Y receptors change as a consequence of seizures. It appears that the predominant change is an increase in Y2 receptors. This would be likely to enhance the ability of neuropeptide Y to inhibit glutamatergic transmission, because Y2 receptors are normally responsible for this effect.65,226
However, other receptors do not necessarily show robust changes, or the studies to date are not in complete agreement.12 An increase in receptors may not lead to a change in effect if neuropeptide Y is not released in sufficient concentration to activate the new receptors.
To add to the complex picture, granule cells formed after seizures, although likely to express neuropeptide Y, have distinct physiological properties and may therefore release neuropeptide Y differently. On the other hand, neurons in the epileptic brain likely release more neuropeptide Y. This may be the case for newly-formed granule cells, which typically exhibit burst discharges181 and therefore may release neuropeptide Y.
In summary, a series of experimental studies have documented the robust influence of neuropeptide Y in the normal dentate gyrus and suggested that this peptide be considered an endogenous anticonvulsant. Moreover, neuropeptide Y expression is highly plastic and altered by seizures. These changes appear to indicate anticonvulsant properties and a compensatory inhibitory role for neuropeptide Y in epilepsy. However, the complexities of neuropeptide Y changes after seizures, and the lack of a detailed understanding of several of these changes, suggest that firm conclusions are still premature.
Somatostatin
Somatostatin is another example of a neuropeptide with robust actions in the dentate gyrus, making it another candidate to serve an endogenous anticonvulsant role.20,223 Normally, somatostatin is preferentially expressed in a subset of GABAergic neurons in the dentate gyrus, which innervate the outer molecular layer and have collaterals in the hilus (Fig. 2A). The axonal projection to the outer molecular layer has received greatest attention because it is most dense, and because of the potential for selective modulation of the lateral perforant path, a major cortical input to the dentate gyrus, which terminates in the outer molecular layer.
In most but not all cases, somatostatin appears to depress this input when single afferent stimuli are tested.14,178 More robust effects are observed after tetanization, which would
normally lead to long-term potentiation (LTP); somatostatin blunts this effect.14 These data suggest that somatostatin depresses the glutamatergic activation of granule cells by an action at the site of synaptic input, and it does so preferentially after high-frequency input. In addition, somatostatin depresses calcium-mediated action potentials (calcium “spikes”) in granule cells by inhibiting N-type calcium channels. The net effect could contribute to the depression of LTP in the lateral perforant path.14 In light of these results, it is no surprise that somatostatin inhibits seizures in animal models of epilepsy.20 Likewise, in somatostatin knockout mice, seizures elicited by chemoconvulsants are more severe, and after discharges are longer in animals that are kindled by stimulation of the perforant path.29
normally lead to long-term potentiation (LTP); somatostatin blunts this effect.14 These data suggest that somatostatin depresses the glutamatergic activation of granule cells by an action at the site of synaptic input, and it does so preferentially after high-frequency input. In addition, somatostatin depresses calcium-mediated action potentials (calcium “spikes”) in granule cells by inhibiting N-type calcium channels. The net effect could contribute to the depression of LTP in the lateral perforant path.14 In light of these results, it is no surprise that somatostatin inhibits seizures in animal models of epilepsy.20 Likewise, in somatostatin knockout mice, seizures elicited by chemoconvulsants are more severe, and after discharges are longer in animals that are kindled by stimulation of the perforant path.29
Somatostatin may also have other actions, however. Thus, in other areas of the hippocampus, exposure to somatostatin leads to additional effects, such as modulation of the M-current.188 This action may have numerous consequences, because M channels are located in diverse areas of the dentate gyrus—not only on neuronal somata, but also on their axons.
As is the case of neuropeptide Y, somatostatin expression is dramatically changed after seizures. The decrease in somatostatin is due to the fact that somatostatin-containing neurons are extremely vulnerable to seizure-induced neuronal death (Fig. 2B). The loss of somatostatin may contribute to the change in hippocampal excitability following seizures.
However, somatostatin cell loss appears to be selective for animal models of epilepsy in which seizures are severe (i.e., models that use an initial period of status epilepticus to induce chronic seizures). Somatostatin cell loss is also evident in hippocampal tissue resected from patients with intractable TLE.167 In contrast, animal models in which seizures are milder, such as electroconvulsive shock or kindling, do not necessarily result in the loss of somatostatinergic neurons, but lead to an increase in somatostatin expression within those neurons that normally express this neuropeptide.153,186
It is also necessary to consider that somatostatin receptors may be altered in epilepsy. In tissue resected from patients with pharmacoresistant TLE, only type 2 (i.e., the receptor known to mediate anticonvulsant effects) is altered in the dentate gyrus.47 It appears that both the mRNA for the type 2 receptor and receptor binding increase in the granule cell layer, remain unaltered in the inner molecular layer, and decrease in the outer molecular layer. The latter changes may reflect a compensation for the loss of afferents, which degenerate due to the vulnerability of hilar somatostatin neurons. Other somatostatin receptors (types 1–4) in the dentate gyrus remain remarkably normal after kainic acid (KA)-induced status epilepticus, although changes occur elsewhere in the hippocampus.162
Taken together, the source of somatostatin and its receptor-mediated actions differ substantially between the normal and the epileptic brain. These differences are likely to alter the peptide’s effects in a pathologic situation. This implies, for example, that synthetic somatostatin analogs may not be able to depress perforant path transmission in the epileptic brain because of the lack of appropriate receptor targets (see earlier discussion), and that it may be possible to design somatostatin-related therapeutic interventions that are geared specifically to the epileptic condition.
The changes in neuropeptide Y and somatostatin in the rat dentate gyrus after chronic seizures are illustrated schematically in FIGURE 2. As noted earlier, neuropeptide Y and somatostatin are just two of a long list of neuropeptides that have been studied intensively in the dentate gyrus in the context of seizures (Table 1). Thus, many neuropeptides appear to modulate excitability normally and specifically influence seizures/epilepsy in animal models of epilepsy. Most of them also have the same dual relationship with seizures as neuropeptide Y and somatostatin (i.e., they are both regulated by seizures and capable of modulating seizures). Finally, it should also be noted that there appear to be significant species differences in neuropeptide expression in the dentate gyrus. However, despite the fact that some of the peptides present in humans are not identical to those in rats or mice, the relationship of peptidergic neurons to epilepsy appears to be essentially similar in rodents and humans.
Calcium-Binding Proteins
Calcium-binding proteins have also received considerable attention with respect to their influence on the function of neurons in the dentate gyrus. Strictly speaking, this group has many members (Table 2), but those in the dentate gyrus that have received most attention are those that have the highest levels of expression. One example is calbindin D28K, which is primarily expressed in granule cells, although studies have also shown immunoreactivity in selected GABAergic neurons.193 Other examples of calcium-binding proteins include parvalbumin and calretinin, which have been selectively localized to GABAergic neurons.198
The distinct distribution of these proteins naturally raises questions about their specific functions in various cell types. Interest grew after it was shown that those neurons in the dentate gyrus that appear most prone to seizure-induced damage (i.e., somatostatin-containing neurons and the glutamatergic mossy cells) lacked either calbindin or parvalbumin. However, later studies suggested a more complex relationship between calcium-binding proteins and vulnerability, because calcium-binding proteins such as calretinin were also found in susceptible neurons.78
Functional studies revealed that calbindin and parvalbumin have important roles in the regulation of calcium within the cell. In the granule cell, calbindin modulates calcium levels and therefore has several potential functions, including an influence on transmitter release. Indeed, overexpression of calbindin leads to alteration in granule cell transmission to pyramidal cells of CA3 due to a presynaptic mechanism at granule cell boutons. These changes are clearly important because of the dramatic alteration in hippocampal function in vivo after overexpression of the protein only in granule cells.57 Parvalbumin also appears to have important functional roles in the regulation of calcium entry, primarily presynaptically.40 These are likely to be substantial in their net effect in vivo, given that parvalbumin knockout mice have altered seizure susceptibility.185 It is not clear, however, whether this is a specific defect in normal parvalbumin function or a compensatory effect.
In human tissue derived from patients with intractable TLE, calbindin expression in granule cells is reduced,131 and the expression of parvalbumin immunoreactivity undergoes complex changes depending on hippocampal subregion and clinical features.198,233 Some argue that these changes may be neuroprotective if intracellular calcium levels were effectively lowered by calcium-binding proteins, but others would suggest that this might not necessarily be the case.219 In summary, the functional significance of neuromodulation by calbindin, parvalbumin, and many other calcium-binding proteins in epilepsy is still controversial and requires careful additional investigation and analysis.35
Table 2 Calcium-binding proteins | |
---|---|
|
Endogenous Trace Metals
Trace metals exert a number of biologic effects throughout the body that are caused, among other mechanisms, by the metals’ ability to serve as cofactors to a large number of enzymes. They also interact directly with cell membrane and intracellular receptors, and regulate oxidation/reduction processes within
cells. Several of these properties play a role in the metals’ effects on cellular excitability in the CNS. It is therefore not surprising that metals such as iron, manganese, and selenium, acting in a neuromodulatory role, may influence the development or termination of seizure activity.85,189,208
cells. Several of these properties play a role in the metals’ effects on cellular excitability in the CNS. It is therefore not surprising that metals such as iron, manganese, and selenium, acting in a neuromodulatory role, may influence the development or termination of seizure activity.85,189,208
The endogenous trace metal zinc has been most frequently associated with seizures. This is primarily due to the observation that zinc is localized in, and can be released from, many glutamatergic neurons throughout the limbic system. Granule cells in the dentate gyrus, and especially their axon terminals, contain a particularly high concentration of zinc. Within the cell, zinc is bound to specific proteins, such as metallothioneins, or exists in presynaptic vesicles and can be released into the extracellular compartment. Extracellularly, zinc can have quite diverse effects, making it difficult to predict its net function. Thus, zinc can depress (e.g., by reducing NMDA receptor function) or enhance (e.g., by interfering with GABAA receptor-mediated inhibition) excitability.76
The inhibition of GABAA receptor function by zinc is particularly interesting in the context of epilepsy. The metal normally has little effect on GABAA receptors on granule cells, because the receptor subunits are not assembled in a combination that optimizes zinc sensitivity. However, these subunits change their expression patterns under epileptic conditions, resulting in reductions in the α1- and γ2-subunits and an increase in the δ-subunit; these changes greatly enhance zinc sensitivity of the receptor.3,42,174
Zinc may also show increased effects on GABAA receptors in the epileptic brain, because zinc-rich mossy fibers develop collaterals that innervate the proximal dendritic region of granule cells (mossy fiber sprouting). These new collaterals constitute an increased source of zinc, which may be of functional significance, because enhanced zinc release from sprouted mossy fibers may further decrease the inhibition of granule cells. In addition, the release of zinc may be greater under conditions of chronic epilepsy, given the predisposition for burst discharges.30 Such a dampening of the normal inhibitory “gate” function of the dentate gyrus might facilitate seizure activity in limbic circuits. Thus, zinc appears to play a critical role in the mechanisms that link changes in GABAA receptor subunits and mossy fiber reorganization in the epileptic brain to the epileptic state.
Cytoskeletal Proteins
Cytoskeletal elements are a fundamental component of nerve cells, and recent studies suggest relevance to epilepsy, particularly for the filamentous proteins. This group includes actin filaments, intermediate filaments (e.g., neurofilaments), and microtubules (such as α- and β- tubulin). In addition to this group, proteins such as clathrin and stathmin are important to consider, because they are critical to endocytosis.
Recent evidence suggests that several of these proteins may also be involved in epileptogenesis, in the response of the nervous system to seizures and, in the developing brain, in the resistance to seizure-induced neuronal damage.126 Notably, some of the seizure-related changes in the expression pattern of cytoskeletal proteins, which may in part be due to cell swelling, have been revealed using gene profiling techniques.127
The involvement of cytoskeletal elements in epilepsy is probably related to their role in the intracellular movement of proteins into different cellular compartments, which, in turn, can modify neuronal excitability. Thus, cytoskeletal proteins are involved in the trafficking of neurotransmitter receptors. Proteins such as clathrin may alter excitability by changing the concentration of molecules available to the extracellular milieu. In addition, the cytoskeleton may be causally involved
in the dendritic deformation that has been described in animals with chronic seizures and in humans with TLE. For example, electron microscopic analysis indicates that cytoskeletal changes may be responsible for the unique beading of dendrites in epileptic tissue,222 although other hypotheses that are independent of the cytoskeleton have also been suggested.207
in the dendritic deformation that has been described in animals with chronic seizures and in humans with TLE. For example, electron microscopic analysis indicates that cytoskeletal changes may be responsible for the unique beading of dendrites in epileptic tissue,222 although other hypotheses that are independent of the cytoskeleton have also been suggested.207
Growth Factors
The term “growth factor” is defined loosely here to refer to several protein families, expressed either in neurons or glia, that were originally identified for their roles in CNS growth and development (Table 3). Subsequent studies revealed that these same proteins influence neurons after maturity, and, interestingly, have striking effects in the context of epilepsy.
Growth factors influence excitability in the adult CNS both directly and indirectly.180 Many of the effects are due to modulation by transcription factors, which in turn affect the expression of proteins that can alter excitability. Their importance to epilepsy is supported by studies showing that the expression of growth factors and their receptors is dramatically altered by seizures, both in animal models of epilepsy and in TLE.180 Similar to the neuropeptide changes observed after seizures (see previous section), these effects may be compensatory in nature and may recapitulate developmental programs—a plausible interpretation in light of the prominent role of growth factors in brain development.
Experimental interference with growth factor function can result in the withering or retraction of axonal pathways, suggesting that these proteins regulate axonal growth and maintain neuronal integrity.206 Furthermore, growth factors influence the morphology and density of dendritic spines,67,111 and also cause additional structural alterations of synapses.228 All these effects may be critical in epilepsy, in which dramatic spine changes occur as a consequence of seizures,213,235 and the axons of injured neurons degenerate. In addition, growth factors may contribute to circuit rearrangements after seizures, including axonal sprouting, formation of new synapses, and other structural alterations.100
Several families of growth factors can be categorized in many ways. In Table 3, the classic growth factor families are organized according to their receptors, which are primarily receptor tyrosine kinases or serine threonine kinases. These include the tyrosine kinase receptor superfamily (ephrins, epidermal growth factor [EGF] family, fibroblast growth factor [FGF] family, insulin growth factor [IGF] family, neurotrophins, and vascular endothelial growth factor [VEGF] family), and the serine threonine kinase receptor superfamily (including transforming growth factor [TGF]-β family). In addition, Table 3 includes two other categories critical to normal growth and development. These include axon guidance molecules (netrins, the reticulon family, semaphorins, and slit proteins) and morphogens (bone-morphogenic proteins, hedgehog family, wnt family). Inflammatory cytokines (interleukins [IL] and tumor necrosis factor [TNF] family) are discussed in Chapter 25. Chemotactic cytokines (chemokines) are also relevant, particularly in relation to the mechanisms that control axon guidance, but to date limited evidence points to their role in seizures, excitability, and epilepsy.
Neurotrophins
The neurotrophins are a family of growth factors that show robust expression in the adult CNS and are known to influence a wide variety of normal functions. They also provide some of the best examples of growth factors that have been shown to influence seizures. The dentate gyrus is useful as an example of a site in which neurotrophins are likely to affect seizure activity, because of the evidence that this region shows robust neurotrophin expression and action. Most of these studies are focused on brain-derived neurotrophic factor (BDNF) or NT-3, and much less is known about the other neurotrophins, (e.g., the prototypic member of the neurotrophin family, NGF, and the fourth major member, NT-4/5).
Figure 3 depicts the normal expression pattern of BDNF in the rodent dentate gyrus. The same pattern appears to be present in humans.148 Thus, BDNF is mainly localized in granule cells, although a small proportion may also be contained in nongranule cells and in afferents from the entorhinal cortex (i.e., the perforant path).41,231,242 Notably, BDNF enhances the expression of neuropeptides such as neuropeptide Y in GABAergic neurons, indicating potentially significant interactions between hippocampal neuromodulators.134 Simply viewed, neuropeptide Y induction may limit excessive excitation by BDNF and thus prevent the development of seizures.
BDNF not only supports dendritic structure and plasticity in the dentate gyrus, but also stimulates the proliferation of cells in the subgranular zone, a major source of newly-generated dentate granule cells in the adult brain.175 Moreover, BDNF has robust effects on the physiology of granule cells and their targets, influencing glutamatergic and GABAergic circuits.24,116,151 These effects often involve changes in transmitter release and depend on protein synthesis.24 In addition, BDNF depolarizes granule cells by an effect on the Nav 1.9 sodium channel.116 Finally, BDNF may also signal via glial cells.168
Table 3 Growth Factors and Cytokines | |||||||||||||||||||||||||||||||||||||||||||||||||||||||||||||||||||||||||||||||||||||||||||||||||||||||||||||||||||||||||||||||||||||||||||||||||||||||||||||||||||||||||||||||||||||||||||||||||||||||||||||||||||||||||||||||||||||||||||||||||||||||||||||||||||||||||||||||||||||||||||||||||||||||||||||||||||||||||||||||||||||||||||||||||||||||||||||||||||||||||||||||||||||||||||||||||||||
---|---|---|---|---|---|---|---|---|---|---|---|---|---|---|---|---|---|---|---|---|---|---|---|---|---|---|---|---|---|---|---|---|---|---|---|---|---|---|---|---|---|---|---|---|---|---|---|---|---|---|---|---|---|---|---|---|---|---|---|---|---|---|---|---|---|---|---|---|---|---|---|---|---|---|---|---|---|---|---|---|---|---|---|---|---|---|---|---|---|---|---|---|---|---|---|---|---|---|---|---|---|---|---|---|---|---|---|---|---|---|---|---|---|---|---|---|---|---|---|---|---|---|---|---|---|---|---|---|---|---|---|---|---|---|---|---|---|---|---|---|---|---|---|---|---|---|---|---|---|---|---|---|---|---|---|---|---|---|---|---|---|---|---|---|---|---|---|---|---|---|---|---|---|---|---|---|---|---|---|---|---|---|---|---|---|---|---|---|---|---|---|---|---|---|---|---|---|---|---|---|---|---|---|---|---|---|---|---|---|---|---|---|---|---|---|---|---|---|---|---|---|---|---|---|---|---|---|---|---|---|---|---|---|---|---|---|---|---|---|---|---|---|---|---|---|---|---|---|---|---|---|---|---|---|---|---|---|---|---|---|---|---|---|---|---|---|---|---|---|---|---|---|---|---|---|---|---|---|---|---|---|---|---|---|---|---|---|---|---|---|---|---|---|---|---|---|---|---|---|---|---|---|---|---|---|---|---|---|---|---|---|---|---|---|---|---|---|---|---|---|---|---|---|---|---|---|---|---|---|---|---|---|---|---|---|---|---|---|---|---|---|---|---|---|---|---|---|---|---|---|---|---|---|---|---|---|---|---|---|---|---|---|---|---|---|---|---|---|---|---|---|---|---|---|---|---|---|---|---|---|---|---|---|---|---|---|---|---|---|
|
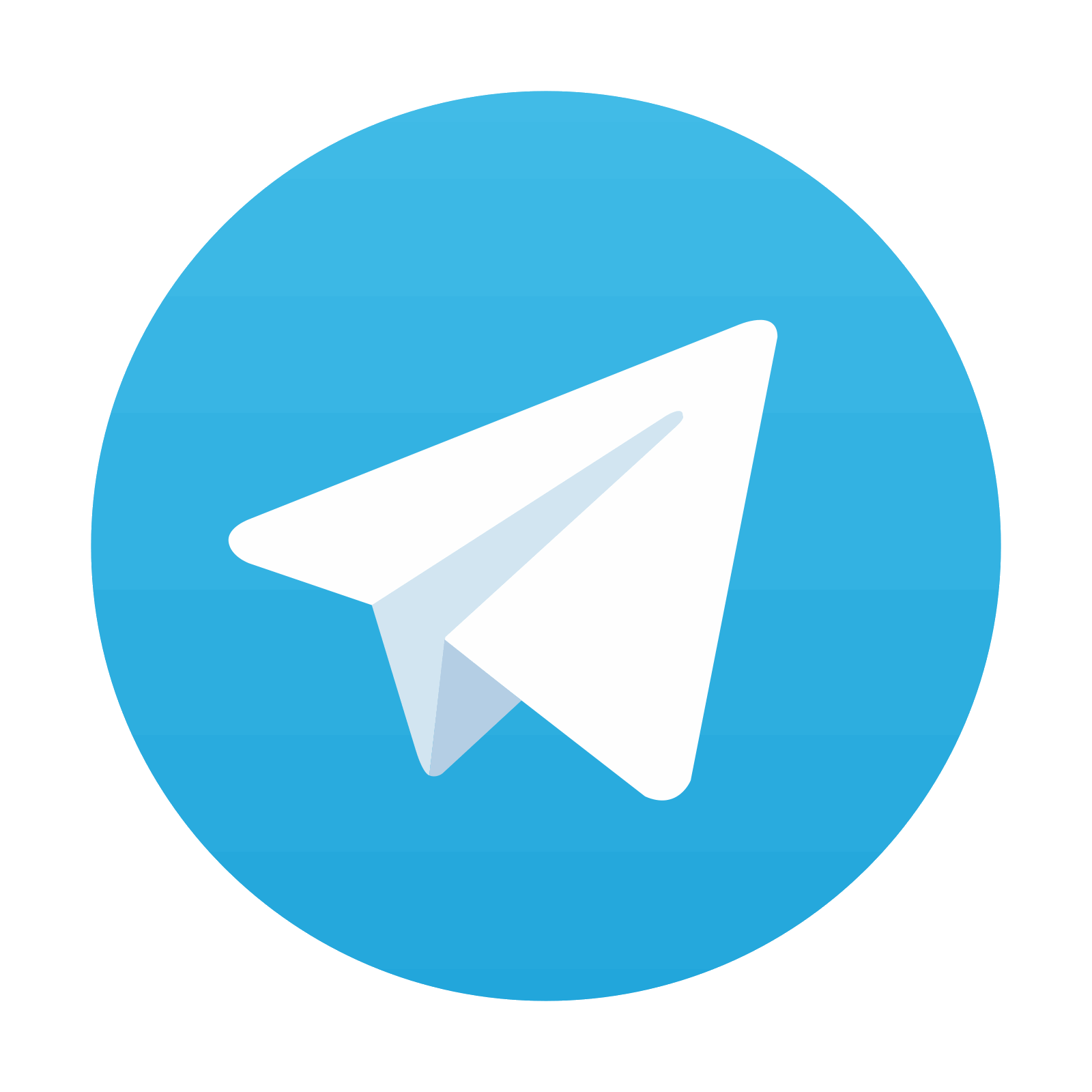
Stay updated, free articles. Join our Telegram channel
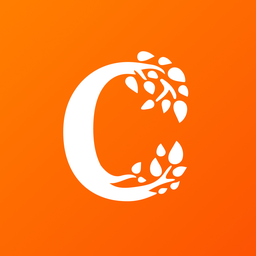
Full access? Get Clinical Tree
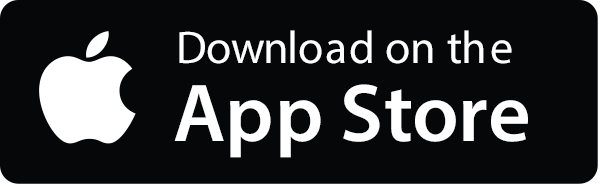
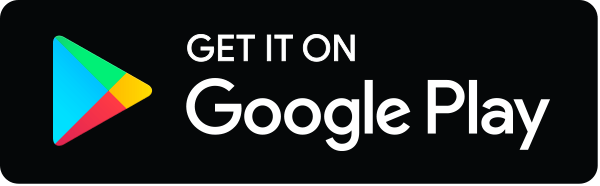
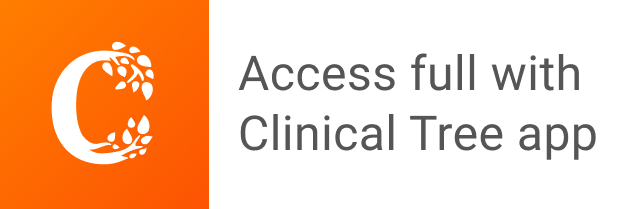