Introduction
Doppler ultrasonography is a technique to measure the velocity and pulsatility of blood flow within the intracranial and the extracranial arteries. Transcranial Doppler (TCD) ultrasonography is frequently used for the clinical evaluation of cerebral vasospasm following subarachnoid hemorrhage (SAH), to evaluate cerebral autoregulation (CA), detect major arterial occlusion, monitor recanalization in acute stroke, monitor cerebral circulation during major cardiovascular procedures, and assess hemodynamics in patients with intracranial hypertension among other indications. Technologic advances such as transcranial color-coded duplex sonography (TCCS) have made TCD more reliable and accurate in its detection of hemodynamic abnormalities. Newer applications of this technology include therapeutic use of ultrasound in the acute stroke setting.
Technical Aspects of Transcranial Doppler
Basics of Ultrasound
Ultrasonic waves that enter human tissue are transmitted, absorbed, reflected, and scattered. Transmission properties of a tissue depend on its density and elasticity. Density and speed of propagation of ultrasound (US) waves determine a tissue’s acoustic impedance. The larger the difference in acoustic impedance between tissues, the more US waves are reflected. Reflection further depends on the angle of insonation, and stronger echoes are received when the angle of insonation is zero. Structures imaged by US B-mode, the two-dimensional visualization of the internal organs and structures on the gray-scale image, are displayed proportionally to the intensity of returning echoes.
Transcranial Doppler and TCCS Techniques
Structures imaged by B-mode are displayed proportionally to the intensity of returning echoes, whereas flow velocity is detected in Doppler mode if there is a difference in frequency between emitted and returning echoes. The magnitude of the frequency shift (ΔF) depends on the ultrasound transmission velocity in the insonated tissue (C), the relative velocities of the reflector (blood, V), and the frequency of the source (Fo). The observed frequency shift (ΔF) is ΔF = 2 VFo/C. The shift is measured only for that component of motion occurring along the axis of the ultrasound beam. Therefore absolute velocity measurements require that a correction be made for the angle () between the vessel and the beam as follows: V = ΔFC/(2 Fo cos ). In pulsed wave Doppler, a transducer generates ultrasound pulses and detects returning echoes. Assuming that the speed of transmission of ultrasound in human tissues is a constant, the time delay between the emitted pulse and the returning echo enables the sampled structure’s depth to be determined. The pulse’s duration and repetition frequency impose limits on the maximum velocity that can be measured. Anatomy is not displayed, however. Identification of intracranial arteries in conventional TCD relies therefore on the a priori defined depths of the sample volume and the direction of flow.
Duplex Doppler imaging combines pulsed-wave Doppler with two-dimensional, real-time B-mode imaging, which allows precise placement of the sample volume in the vessel. Optimal angle correction for velocity calculations can be performed, as the course of the vessel in relation to the US beam is visually depicted. Color-coded duplex sonography is the most commonly used technology today for carotid imaging and TCCS ( Fig. 30.1 ). Red indicates blood flow toward the transducer, whereas blue represents flow away from the transducer. High-flow velocities are depicted with increasing brightness, thus the presence, direction, and flow disturbances can be quickly identified.

The insonation angle is important in flow velocity measurements with TCCS because trajectory of a particular vessel in the intracranial space is unpredictable. Thus if the velocity measurements are adjusted for the angle, the velocity values are closer to the true values because the vessel’s geometric factor is accounted for. If a measured velocity is 170 cm/second and the angle is 20 degrees, the true velocity is 181 cm/second; however, if the angle is 40 degrees, the true velocity is 222 cm/second. Such difference, caused by erroneous flow velocity measurement, can change management of a patient. TCD/TCCS cannot be performed in about 11% of patients because the acoustic window in the temporal bone is weak or absent. Also following a pterional craniotomy the acoustic window may be degraded because of subtemporal bone work or the presence of Gelfoam in the epidural space. Frequencies used for neurosonology range between 1 and 3.5 MHz for TCD/TCCS and 7.5 and 16 MHz for carotid imaging.
Bioeffects and Safety of Ultrasound
Diagnostic US can produce heat that may be hazardous to sensitive organs. Nonthermal effects, such as pressure changes and mechanical disturbances, in tissue have not been demonstrated in humans. US used in therapy, however, can cause both substantial temperature increase and mechanical damage in the tissue.
Thermal Effects
Denser tissue absorbs more heat from US, thus fluid does not heat very much, whereas bone heats the most, and on bone surface the heat accumulation can be up to 50 times faster than in soft tissues. This heating effect can be of importance, particularly with use of long-term TCD monitoring and its therapeutic applications. The thermal index (TI), provided in US machines estimates the risk from heat. When the TI is greater than 1, the risks of US should be weighed against the benefits. The exposure has to be minimized despite reports that short-term monitoring does not increase temperature at the temporal window in vivo.
Nonthermal Effects
US can also produce various mechanical effects such as cavitation, pressure amplitude, force, torque, and acoustic streaming. Cavitation occurs when US passes through an area that contains a cavity, such as a gas bubble. US can cause the bubble to expand and contract rhythmically. When bubbles pulsate, they send secondary US waves in all directions. These secondary waves can actually improve US imaging. If the bubbles contract toward the point of collapsing, they can build up very high temperatures and pressures for a few tens of nanoseconds. These high temperatures and pressures can produce free radicals and other toxic compounds that, although unlikely, could theoretically cause genetic damage. The rapid contraction of bubbles can also cause microjets of liquid that can damage cells. The safety guidelines for diagnostic US are designed to prevent cavitations to occur. US-induced changes in pressure, force, torque, and streaming, in turn, can cause audible sounds, electrical changes in cell membranes that make them more permeable to large molecules, movement and redistribution of cells in liquid, and cell damage. In the experimental setting using promyelocytic leukemia cells, sonoporation may lead to antiproliferation effects including cell-cycle arrest and apoptosis through disrupting various cell signaling pathways. In liquids, US causes a type of stirring action called acoustic streaming . As the acoustic pressure of US increases, the flow of liquid speeds up. When the streaming liquid comes near a solid object, shearing may occur, which can damage platelets and lead to abnormal blood clotting.
Effects of Ultrasound Contrast Agents
These agents usually take the form of stable gas-filled microbubbles (MBs) that can produce cavitations or microstreaming, the risk of which increases with the mechanical index value. Additionally, a radiation force produced by the acoustic wave, considered as noncavitational and nonthermal mechanisms of ultrasonic bioeffects, may act on particles and microbubbles.
Cerebral Vascular Reactivity and Transcranial Ultrasound Testing of Cerebral Blood Flow Regulation
Cerebral Autoregulation
CA is the ability of brain microvasculature to maintain cerebral blood flow (CBF) relatively constant despite wide variations in cerebral perfusion pressure (CPP). In the healthy state CA keeps CBF relatively constant within the range of mean arterial blood pressure (MAP) between 60 and 150 mm Hg. However, the upper and lower limits of CA can be shifted up or down by endogenous as well as exogenous factors. Sympathetic nervous system stimulation and angiotensin II can shift the upper and lower limits of CA up toward higher pressures, whereas antihypertensive medications have opposite effects. Patients who have untreated hypertension have limits of regulation set at a higher level compared with healthy people ; thus overzealous antihypertensive treatment may lead to a dangerous CBF reduction at a relatively high MAP. The mechanisms of CA are complex and not fully understood and appear to be maintained by three different control pathways: vasogenic, metabolic, and neurogenic.
The vasogenic mechanism of CA is based on the intrinsic ability of cerebral vessels to respond to changes in the wall’s shear stress and transluminal pressure. An increase in transluminal pressure activates vascular smooth muscle cells, leading to vasoconstriction, whereas increased shear stress triggers vasodilation. In metabolic regulation, cerebrovascular resistance (CVR) is modified by PaCO 2 , PaO 2 , and release of adenosine and potassium ions from neurons in response to insufficient blood supply. Hypercapnia and the resulting decrease in extracellular pH causes vasodilation; hypocapnia leads to vasoconstriction and CBF decrease. The importance of neurogenic regulation in the control of CBF is a matter of debate. The cerebral vessels are innervated by an extrinsic system such as nerve fibers that originate in ganglia belonging to sympathetic, parasympathetic, and sensory ganglia, and an intrinsic system that is composed from nerves originating in different parts of brain such as the nucleus basalis, locus coeruleus, and raphe nucleus. Sympathetic stimulation constricts large cerebral arteries, but CBF does not decrease because of immediately compensated vasodilation of resistance arterioles. Sympathetic innervation seems to protect the brain against MAP increases because sympathetic activation shifts the upper and lower limits of CA toward a higher MAP. Activation of the parasympathetic vascular nerve fibers causes vasodilation. Vascular fibers belonging to the intrinsic system may modulate vascular tone directly or through stimulation of perivascular interneurons and glial cells. However, the significance of this system is being elucidated.
Assessment of Cerebral Blood Flow Regulation with Ultrasound
The CA can be evaluated by measuring relative blood flow changes in response to the change in the MAP using “static” or “dynamic” approaches. In the static method, CA efficiency (i.e., the overall change in cerebral arterial resistance [CAR] induced by changes in MAP) is assessed under steady-state conditions. In this method the first cerebral blood flow velocity (CBFV) measurement obtained at a constant baseline MAP is followed by another steady-state measurement that is taken after the CAR to a change in MAP. CA functions properly if the MAP change within the lower and upper limits of CA, estimated for healthy humans, does not affect CBFV. Dynamic methods use rapid MAP changes and analyze the instantaneous CBFV in the process. Systemic hemodynamic changes may be induced using rapid leg cuff deflation, progressive lower body negative pressure, the Valsalva maneuver, deep breathing, ergometric exercise, or head-down tilting ( Fig. 30.2 ). Dynamic methods are able to assess both efficiency and latency of the CA response, that is, the overall change in CAR and the time in which the CAR change is achieved. The use of systemic hypotension to challenge the CA response is limited, however, because pharmacologically induced hypotension could result in ischemic injury in patients with already insufficient CBF.

Two commonly used, but somewhat less dynamic methods of physiologic challenge of CA that carry much less risk of ischemia include administration of acetazolamide and systemic PaCO 2 manipulations. Acetazolamide is a carbonic anhydrase inhibitor that acts as a potent vasodilator when administered intravenously in a dose of 15 to 18 mg/kg of body weight (standard dose 1000 mg). Acetazolamide slowly penetrates the blood-brain barrier, where it reversibly inhibits carbonic anhydrase that catalyzes the conversion of bicarbonate and hydrogen ion to water and carbon dioxide (CO 2 ). Acetazolamide decreases the production of bicarbonate and results in a decrease in the extracellular pH in the brain. This induced acidosis results in vasodilation, and CBF increase, whereas MAP, heart rate (HR), and respiratory rate are unaffected. The effect on CBF may be seen within the first 5 minutes after administration. In healthy subjects, peak CBF augmentation of about 30% to 60% occurs at approximately 10 minutes after bolus intravenous administration and changes little over the next 20 minutes ( Fig. 30.3 ). This response can be blunted in an older population. The percentage of increase in CBF after acetazolamide administration may be used to define cerebrovascular reserve as follows:
CVR = ( CBF post − CBF pre ) / CBF preacetazolamide × 100 %

Although a global, symmetric increase in CBF by about 30% is accepted as normal, a variety of criteria are used to define an abnormal response to acetazolamide, including: (1) greater than 5% decrement in absolute CBF, (2) a less than 10% increment in absolute CBF, (3) an absolute change of less than 10 mL/100 g/minute, and (4) a value greater than two standard deviations below control values. Acetazolamide is generally well tolerated in a dose less than 1000 mg. The most commonly reported side effects include transient circumoral numbness, paresthesias, and headaches.
Manipulation of systemic partial CO 2 pressure (PaCO 2 ) also can be used to test CVR. Low-level hypercarbia results in a reproducible CBF increase between 0.01 and 0.02 mL/g/min for each 1 mm Hg PaCO 2 increase. The effect is rapid and quickly reversible. CO 2 manipulation is performed using several techniques, including: (1) breath holding or hyperventilation, (2) re-breathing, or (3) inhalation of 3% to 5% CO 2 . The effect of altered CO 2 tension on CBF is quantified either as a fixed effect for a particular CO 2 manipulation (similar to the quantification of acetazolamide effect) or as the slope of the CO 2 -CBF relationship according to the following formula :
CVR = [ ( CBF post-CO 2 − CBF pre-CO 2 ) / ( C BF pre-CO 2 ) ] × [ 100 / ( Pa pre-CO 2 – Pa post-CO 2 ) ]
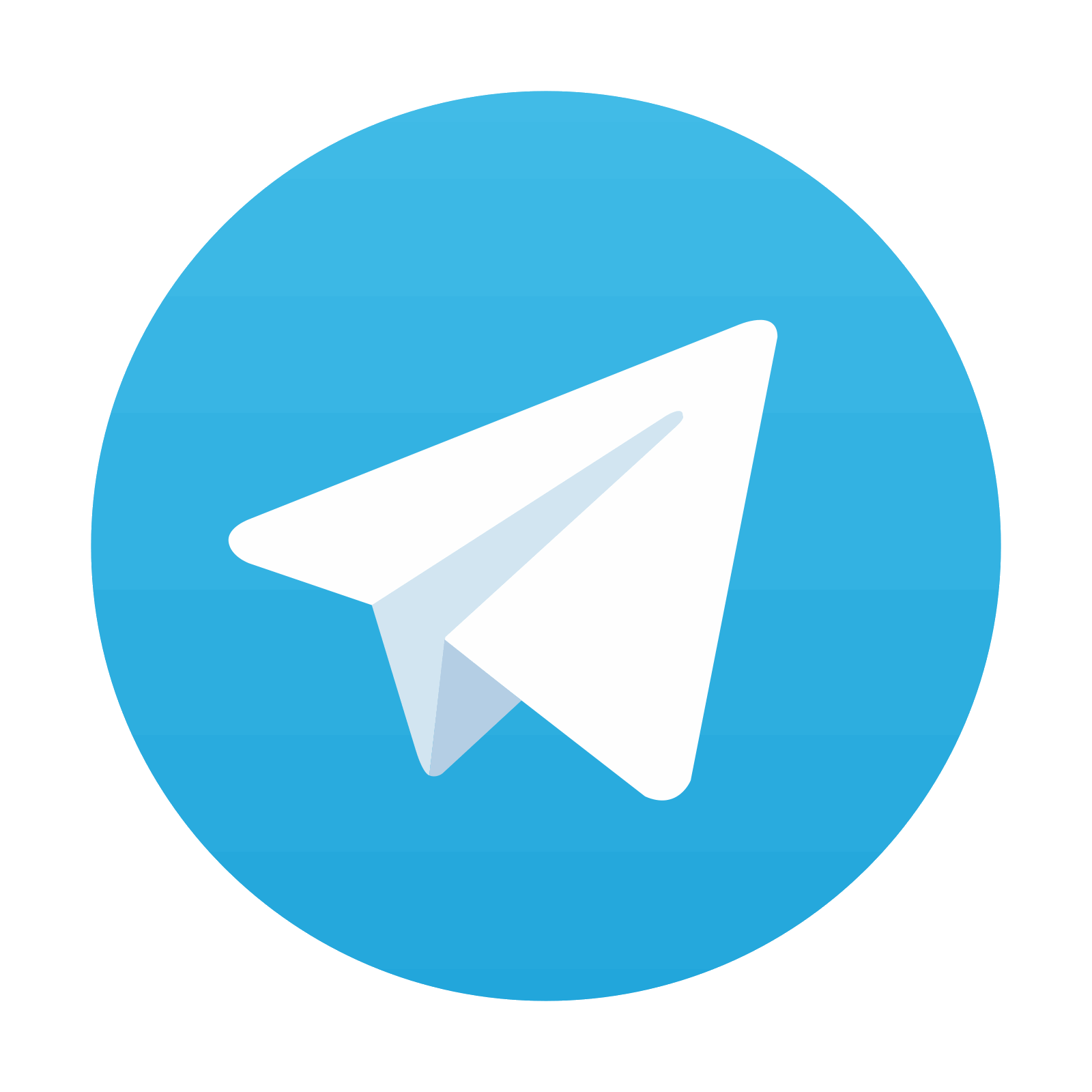
Stay updated, free articles. Join our Telegram channel
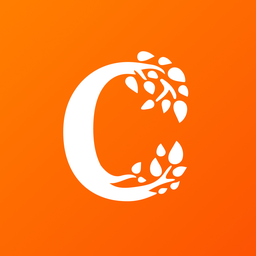
Full access? Get Clinical Tree
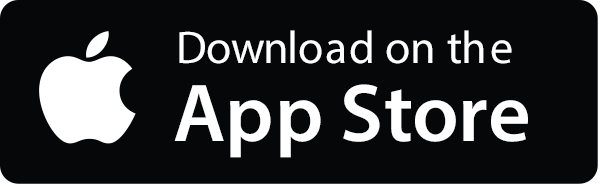
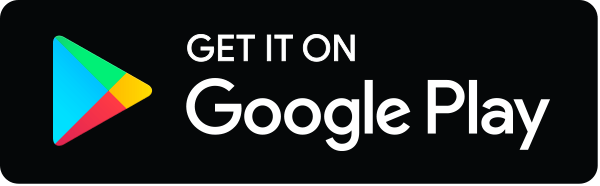