Neurotransmitters
A Chemical Messenger Must Meet Four Criteria to Be Considered a Neurotransmitter
Only a Few Small-Molecule Substances Act as Transmitters
Small-Molecule Transmitters Are Actively Taken Up into Vesicles
Many Neuroactive Peptides Serve as Transmitters
Peptides and Small-Molecule Transmitters Differ in Several Ways
Peptides and Small-Molecule Transmitters Coexist and Can Be Co-released
Removal of Transmitter from the Synaptic Cleft Terminates Synaptic Transmission
CHEMICAL SYNAPTIC TRANSMISSION can be divided into four steps—(1) synthesis and storage of a transmitter substance, (2) release of the transmitter, (3) interaction of the transmitter with receptors at the postsynaptic membrane, and (4) removal of the transmitter from the synaptic cleft. In the previous chapter we considered steps 2 and 3: the release of transmitters and how they interact with postsynaptic receptors. We now turn to the initial and final steps of chemical synaptic transmission: the synthesis of transmitter molecules and their removal from the synaptic cleft after synaptic action.
A Chemical Messenger Must Meet Four Criteria to Be Considered a Neurotransmitter
Before considering the biochemical processes involved in synaptic transmission, it is important to make clear what is meant by a chemical transmitter. The concept is empirical rather than logical and has changed over the years with increased understanding of synaptic transmission.
The concept that nerve stimulation led to release of chemical signals was elaborated as early as 1905 by the physiologist John Newport Langley, who demonstrated that adrenomedullary extracts elicited tissue responses comparable to sympathetic nerve stimulation. However, Thomas Renton Elliott is generally credited with the first experimental evidence of chemical neurotransmission in his observations that the physiological effects of sympathetic nerve stimulation were due to release of adrenaline. In 1921 Otto Loewi demonstrated the release of acetylcholine (ACh) from vagus nerve terminals in frog hearts. Henry Dale extended Loewi’s work on ACh, later sharing the Nobel Prize with Loewi. In 1946 Ulf von Euler reported further work on adrenergic transmission. The terms cholinergic and adrenergic were introduced to indicate that a neuron makes and releases ACh or norepinephrine (or epinephrine), the two substances first recognized as neurotransmitters.
Since that time many other substances have been identified as transmitters. Furthermore, because of the work of Bernard Katz in the 1950s on quantal release (see Chapter 12), it is usually taken for granted that substances acting as transmitters are stored in vesicles at synapses and released by exocytosis. Nevertheless some substances considered to be neurotransmitters are released into the synaptic cleft directly from the cytoplasm as well as by exocytosis. Thus ideas about neurotransmitters have had to be modified continually to accommodate new information about the cell biology of neurons and the pharmacology of receptors.
As a first approximation, a neurotransmitter can be defined as a substance that is released by a neuron and that affects a specific target in a specific manner. A target can be either another neuron or an effector organ, such as muscle or gland. As with many other operational concepts in biology, the concept of a transmitter is not precise. Neurotransmitters are protean, resembling other released agents in many regards yet also differing from them depending on the site of action and circumstances. Although the actions of hormones and neurotransmitters are quite similar, neurotransmitters usually act on targets that are close to the site of transmitter release, whereas hormones are released into the bloodstream to act on distant targets.
Neurotransmitters differ from autacoids in that a transmitter typically acts on a target other than the releasing neuron itself, whereas an autacoid acts only on the cell from which it was released. Nevertheless, at some synapses transmitters activate not only receptors in the postsynaptic cell but also autoreceptors on the presynaptic terminal. Autoreceptors usually modulate synaptic transmission that is in progress, for example, by limiting further release of transmitter or inhibiting subsequent transmitter synthesis. Receptors can also exist on presynaptic terminals at axo-axonic synapses, where the presynaptic terminal receives synaptic input from another neuron. These receptors function as heteroreceptors that regulate terminal excitability and transmitter release (see Chapter 12).
Importantly, the interaction of neurotransmitters with receptors is typically transient, lasting from milliseconds to minutes. Nevertheless, neurotransmitter action can result in long-term changes within target cells lasting hours or days. Lastly, increasing evidence suggests that a non-neural cell, the astrocyte, can also synthesize, store, and release neurotransmitters as well as express receptors that modulate astrocyte function.
Despite these difficulties in arriving at a strict definition, a limited number of substances of low molecular weight are generally accepted as neurotransmitters. Even so, it is often difficult to demonstrate that a specific transmitter operates at a particular synapse, particularly given the diffusibility and rapid reuptake or degradation of transmitters at the synaptic cleft. Because of this difficulty, many neurobiologists believe that a substance should not be accepted as a neuro-transmitter unless the following four criteria are met:
1. It is synthesized in the presynaptic neuron.
2. It is present in the presynaptic terminal and is released in amounts sufficient to exert a defined action on the postsynaptic neuron or effector organ.
3. When administered exogenously in reasonable concentrations it mimics the action of the endogenous transmitter (for example, it activates the same ion channels or second-messenger pathway in the postsynaptic cell).
4. A specific mechanism usually exists for removing the substance from the synaptic cleft.
The nervous system makes use of two main classes of chemical substances for signaling: small-molecule transmitters and neuroactive peptides, which are short polymers of amino acids. Both classes of neurotransmitters are contained in vesicles, large and small. Neuropeptides are packaged in large dense-core vesicles (approximately 70–250 nm in diameter), which release their contents by exocytosis, similar to those seen in secretory glands and mast cells. Small-molecule transmitters are packaged in small electron-lucent vesicles (∼40 nm in diameter), which release their contents through exocytosis at active zones closely associated with specific Ca2+ channels (see Chapter 12). Large dense-core vesicles can contain both small-molecule transmitters and neuropeptides.
Both types of vesicles are found in most neurons but in different proportions. Small synaptic vesicles are characteristic of neurons that use ACh, glutamate, γ-aminobutyric acid (GABA), and glycine as transmitters, whereas large dense-core vesicles are typical of neurons that use catecholamines and serotonin as transmitters. The adrenal medulla, once used as a model for studying exocytosis, contains only secretory granules that are similar to large dense-core vesicles. Because dense-core vesicles can contain both small-molecule transmitters and neuropeptides, they are important in co-transmission, which is discussed later in this chapter.
Only a Few Small-Molecule Substances Act as Transmitters
A relatively small number of low-molecular-weight substances are generally accepted as neurotransmitters, including ACh, amino acids or their amine-containing derivatives, adenosine triphosphate (ATP), and ATP metabolites (Table 13–1). The amine chemical messengers share many biochemical similarities. All are charged small molecules that are formed in relatively short biosynthetic pathways and synthesized either from essential amino acids or from precursors derived from the major carbohydrate substrates of intermediary metabolism. Like other pathways of intermediary metabolism, synthesis of these neurotransmitters is catalyzed by enzymes that, almost without exception, are cytosolic. ATP, which originates in mitochondria, is abundantly present throughout the cell.
Table 13–1 Small-Molecule Transmitter Substances and Their Precursors
As in any biosynthetic pathway, the overall synthesis of amine transmitters typically is regulated at one rate-limiting enzymatic reaction. The rate-limiting step often is characteristic of one type of neuron and usually is absent in other types of mature neurons.
Acetylcholine
Acetylcholine is the only low-molecular-weight amine transmitter substance that is not an amino acid or derived directly from one. The biosynthetic pathway for ACh has only one enzymatic reaction, catalyzed by choline acetyltransferase (step 1 in the reaction shown below). This transferase is the characteristic and limiting enzyme in ACh biosynthesis. Nervous tissue cannot synthesize choline, which is derived from the diet and delivered to neurons through the blood stream. The co-substrate, acetyl coenzyme A (acetyl CoA), participates in many general metabolic pathways and is not restricted to cholinergic neurons.
Acetylcholine is released at all vertebrate neuromuscular junctions by spinal motor neurons (see Chapter 9). In the autonomic nervous system it is the transmitter for all preganglionic neurons and for parasympathetic postganglionic neurons as well (see Chapter 47). Cholinergic neurons form synapses throughout the brain; those in the nucleus basalis have particularly widespread projections to the cerebral cortex. Acetylcholine (together with a noradrenergic component) is a principle neurotransmitter of the reticular activating system, which modulates arousal, sleep, wakefulness, and other critical aspects of human consciousness.
Biogenic Amine Transmitters
The term biogenic amine, although chemically imprecise, has been used for decades to designate certain neurotransmitters. This group includes the catecholamines and serotonin. Histamine, an imidazole, is also often referred to as a biogenic amine, although its biochemistry is remote from the catecholamines and the indolamines.
Catecholamine Transmitters
The catecholamine transmitters—dopamine, norepinephrine, and epinephrine—are all synthesized from the essential amino acid tyrosine in a common biosynthetic pathway containing five enzymes: tyrosine hydroxylase, pteridine reductase, aromatic amino acid decarboxylase, dopamine β-hydroxylase, and phenylethanolamine-N-methyl transferase. Catecholamines have the catechol nucleus, a 3,4-dihydroxylated benzene ring.
The first enzyme, tyrosine hydroxylase (step 1 below), is an oxidase that converts tyrosine to L-dihydroxyphenylalanine (L-DOPA). This enzyme is rate-limiting for the synthesis of both dopamine and norepinephrine. It is present in all cells producing catecholamines and requires a reduced pteridine cofactor, Pt-2H, which is regenerated from pteridine (Pt) by another enzyme, pteridine reductase, which uses nicotinamide adenine dinucleotide (NADH) (step 4 below). This reductase is not specific to neurons.
L-DOPA is next decarboxylated by aromatic amino acid decarboxylase, also called L-DOPA decarboxylase (step 2 below), to yield dopamine and CO2:
The third enzyme in the sequence, dopamine β-hydroxylase (step 3 below), converts dopamine to norepinephrine. Unlike all other enzymes in the biosynthetic pathways of small-molecule neurotransmitters, dopamine β-hydroxylase is membrane-associated. It is bound tightly to the inner surface of aminergic vesicles as a peripheral protein. Consequently, norepinephrine is the only transmitter synthesized within vesicles.
In the central nervous system norepinephrine is used as a transmitter by neurons with cell bodies in the locus ceruleus, a nucleus of the brain stem with many complex modulatory functions (see Chapter 46). Although these adrenergic neurons are relatively few in number, they project diffusely throughout the cortex, cerebellum, and spinal cord. In the peripheral nervous system norepinephrine is the transmitter of the postganglionic neurons in the sympathetic nervous system (see Chapter 47).
In addition to these four catecholaminergic biosynthetic enzymes, a fifth enzyme, phenylethanolamine-N-methyltransferase (step 5 below), methylates norepinephrine to form epinephrine (adrenaline) in the adrenal medulla. This reaction requires S-adenosylmethionine as a methyl donor. The transferase is a cytoplasmic enzyme. Thus, for epinephrine to be formed, its immediate precursor, norepinephrine, must exit from vesicles into the cytoplasm. For epinephrine to be released, it must then be taken up into vesicles. Only a small number of neurons in the brain use epinephrine as transmitter.
The production of these catecholamine neurotransmitters is controlled by feedback regulation of the first enzyme in the pathway. Not all cells that release catecholamines express all five biosynthetic enzymes, although cells that release epinephrine do. During development the expression of the genes encoding these synthetic enzymes is independently regulated and the particular catecholamine produced by a cell is determined by which enzyme(s) in the step-wise pathway are not expressed. Thus, neurons that release norepinephrine do not express the methyltransferase, and neurons that release dopamine do not express the transferase or dopamine β-hydroxylase.
Of the four major dopaminergic nerve tracts, three arise in the midbrain (see Chapter 46). Dopaminergic neurons in the substantia nigra that project to the striatum are important for the control of movement and are affected in Parkinson disease and other disorders of movement. The mesolimbic and mesocortical tracts are critical for affect, emotion, attention, and motivation and are implicated in schizophrenia and drug addiction (see Chapters 48, 49, and 62). A fourth dopaminergic tract, the tuberoinfundibular pathway, originates in the arcuate nucleus of the hypothalamus and projects to the pituitary gland, where it regulates secretion of hormones (see Chapter 46).
The synthesis of biogenic amines is highly regulated and can be rapidly increased. As a result, the amounts of transmitter available for release can keep up with wide variations in neuronal activity. Opportunities for regulating both the synthesis of catecholamine transmitters and the production of enzymes in the stepwise catecholamine pathway are discussed in Box 13-1.
Trace amines, naturally occurring catecholamine derivatives, may also be transmitters. In invertebrates the tyrosine derivatives tyramine and octopamine play key roles in numerous physiological processes including behavioral regulation. Trace amine receptors also have been identified in mammals, where their function is still a matter of some controversy.
Box 13–1 Catecholamine Production Varies with Neuronal Activity
The production of norepinephrine is able to keep up with wide variations in neuronal activity because it is highly regulated. In autonomic ganglia the amount of norepinephrine is regulated transsynaptically. Activity in the presynaptic neurons, which are both cholinergic and peptidergic, first induces short-term changes in second messengers in the postsynaptic adrenergic cells.
These changes increase the supply of norepinephrine through the cAMP-dependent phosphorylation of tyrosine hydroxylase, the first enzyme in the norepinephrine biosynthetic pathway. Phosphorylation enhances the affinity of the hydroxylase for the pteridine cofactor and diminishes feedback inhibition by end products such as norepinephrine. Phosphorylation of tyrosine hydroxylase lasts only as long as cAMP remains elevated, as the phosphorylated hydroxylase is quickly dephosphorylated by protein phosphatases.
If presynaptic activity is sufficiently prolonged, however, other changes in the production of norepinephrine will occur. Severe stress to an animal results in intense presynaptic activity and persistent firing of the postsynaptic adrenergic neuron, placing a greater demand on transmitter synthesis. To meet this challenge, the tyrosine hydroxylase gene is induced to increase production of the enzyme protein. Elevated amounts of tyrosine hydroxylase are observed in the cell body within hours after stimulation and at nerve endings days later.
This induction of increased levels of tyrosine hydroxylase begins with the persistent release of chemical transmitters from the presynaptic neurons and prolonged activation of the cAMP pathway in postsynaptic adrenergic cells, which activates the cAMP-dependent protein kinase (PKA). This kinase phosphorylates not only existing tyrosine hydroxylase molecules, but also a transcription factor, cAMP response element binding protein (CREB).
Once phosphorylated, CREB binds a specific DNA enhancer sequence called the cAMP-recognition element (CRE), which lies upstream (5′) of the gene for the hydroxylase. Binding of CREB to CRE facilitates the binding of RNA polymerase to the gene’s promoter, increasing tyrosine hydroxylase transcription. Induction of tyrosine hydroxylase was the first known example of a neurotransmitter altering gene expression.
There is a high degree of similarity in amino acid and nucleic acid sequences encoding three of the biosynthetic enzymes: tyrosine hydroxylase, dopamine β-hydroxylase, and phenylethanolamine-N-methyltransferase. This similarity suggests that the three enzymes arose from a common ancestral protein.
Moreover, long-term changes in the synthesis of these enzymes are coordinately regulated in adrenergic neurons. At first, this discovery suggested that the genes encoding these enzymes might be located sequentially along the same chromosome and be controlled by the same promoter, like genes in a bacterial operon. But in humans the genes for the biosynthetic enzymes for norepinephrine are not located on the same chromosome. Therefore, coordinate regulation is likely achieved by parallel activation through similar but independent transcription activator systems.
Serotonin
Serotonin (5-hydroxytryptamine or 5-HT) and the essential amino acid tryptophan from which it is derived belong to a group of aromatic compounds called indoles, with a five-member ring containing nitrogen joined to a benzene ring. Two enzymes are needed to synthesize serotonin: tryptophan (Trp) hydroxylase (step 1 in the following reaction), an oxidase similar to tyrosine hydroxylase, and aromatic amino acid decarboxylase, also called 5-hydroxytryptophan (5-HTP) decarboxylase (step 2 in the following reaction).
The limiting reaction is catalyzed by the first enzyme in the pathway, tryptophan hydroxylase. Tryptophan hydroxylase is similar to tyrosine hydroxylase not only in catalytic mechanism but also in amino acid sequence. The two enzymes are thought to stem from a common ancestral protein by gene duplication because the two hydroxylases are syntenic, that is, they are encoded by genes close together on the same chromosome (tryptophan hydroxylase, 11p15.3-p14; tyrosine hydroxylase, 11p15.5). The second enzyme in the pathway, 5-hydroxytryptophan decarboxylase, is identical to L-DOPA decarboxylase. Enzymes with similar activity, L-aromatic amino acid decarboxylases, are present in non-nervous tissues as well.
The cell bodies of serotonergic neurons are found in and around the midline raphe nuclei of the brain stem and are involved in regulating attention and other complex cognitive functions (Chapter 46). The projections of these cells (like those of noradrenergic cells in the locus ceruleus) are widely distributed throughout the brain and spinal cord. Serotonin and the catecholamines norepinephrine and dopamine are implicated in depression, a major mood disorder. Antidepressant medications inhibit the uptake of serotonin, norepinephrine, and dopamine, thereby increasing the magnitude and duration of the action of these transmitters, which in turn leads to altered cell signaling and adaptations (see Chapter 63).
Histamine
Histamine, derived from the essential amino acid histi-dine by decarboxylation, contains a characteristic five-member ring with two nitrogen atoms. It has long been recognized as an autacoid, active when released from mast cells in the inflammatory reaction and in the control of vasculature, smooth muscle, and exocrine glands (eg, secretion of highly acidic gastric juice). Histamine is a transmitter in both invertebrates and vertebrates. It is concentrated in the hypothalamus, one of the centers for regulating the secretion of hormones (see Chapter 47). The decarboxylase catalyzing its synthesis (step 1 below), although not extensively analyzed, appears to be characteristic of histaminergic neurons.
Amino Acid Transmitters
In contrast to acetylcholine and the biogenic amines, which are not intermediates in general metabolic pathways and are produced only in certain neurons, the amino acids glutamate and glycine are not only neurotransmitters but also universal cellular constituents. Because they can be synthesized in neurons, neither are essential amino acids.
Glutamate, the neurotransmitter most frequently used at excitatory synapses throughout the central nervous system, is produced from α-ketoglutarate, an intermediate in the tricarboxylic acid cycle of intermediary metabolism. After it is released, glutamate is taken up from the synaptic cleft by specific transporters in the membrane of both neurons and glia (see below). The glutamate taken up by astrocytes is converted to glutamine by the enzyme glutamine synthase. This glutamine then diffuses back into neurons that use glutamate as a transmitter, where it is hydrolyzed back to glutamate. Phosphate-activated glutaminase (PAG), which is present at high concentrations in these neurons, is responsible for salvaging the molecule for reuse as a transmitter.
Glycine is the major transmitter used by inhibitory interneurons of the spinal cord. It is also an allosteric modulator of the N-methyl-D-aspartate (NMDA) subtype of glutamate receptors (see Chapter 10). Glycine is synthesized from serine. Its specific biosynthesis in neurons is not well understood, but its biosynthetic pathway in other tissues is well known. The amino acid GABA is synthesized from glutamate in a reaction catalyzed by glutamic acid decarboxylase (step 1 below):
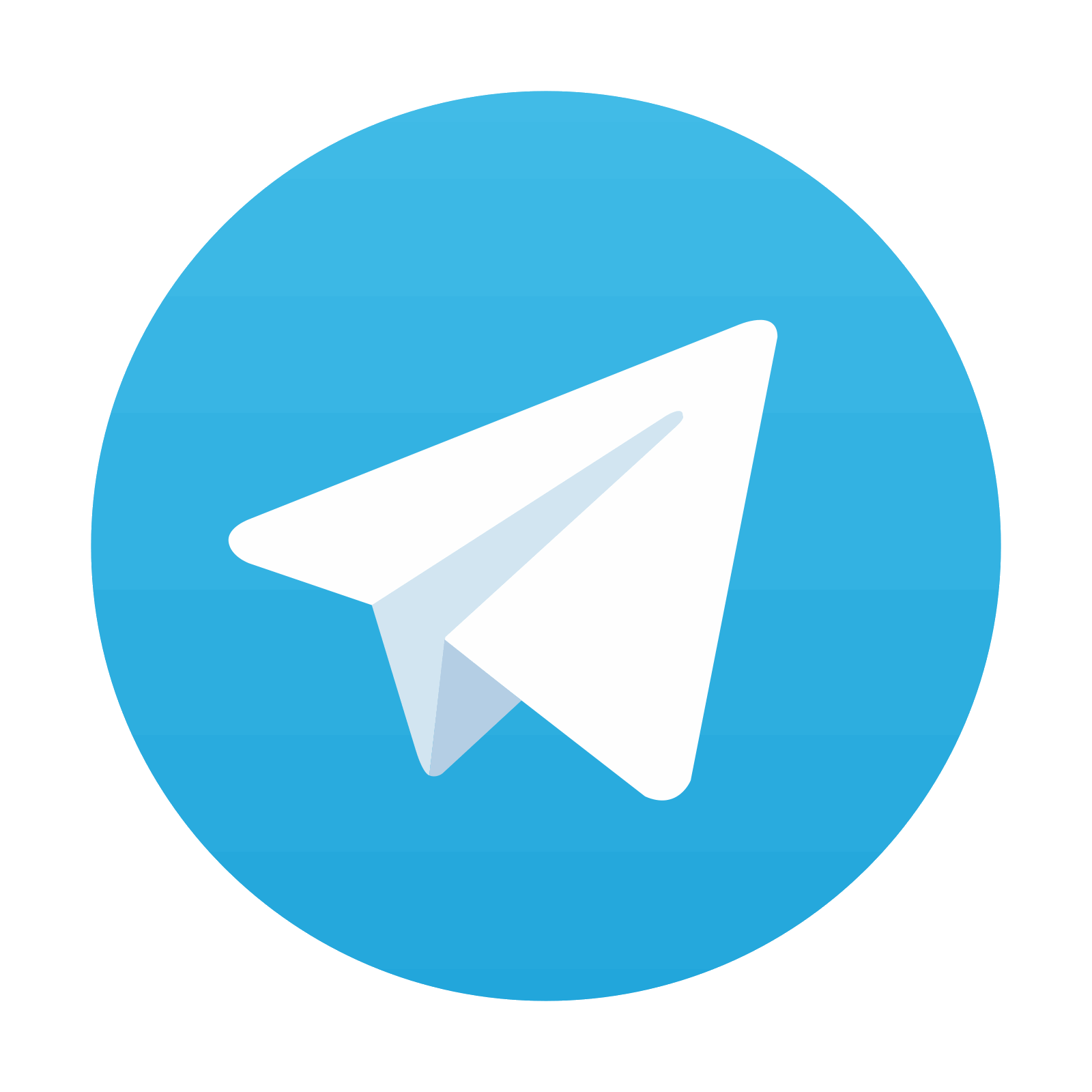
Stay updated, free articles. Join our Telegram channel
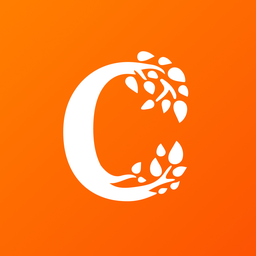
Full access? Get Clinical Tree
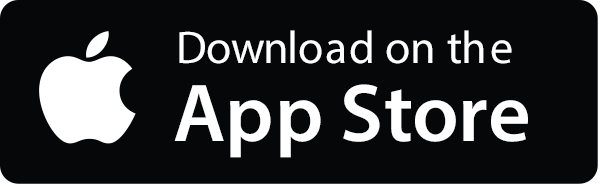
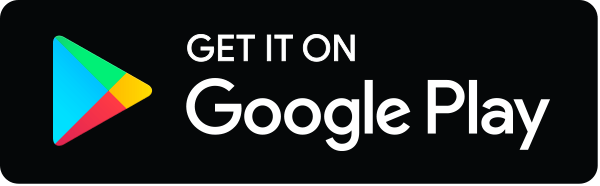