© Springer Science+Business Media New York 2016
Fredric P. Manfredsson (ed.)Gene Therapy for Neurological DisordersMethods in Molecular Biology138210.1007/978-1-4939-3271-9_2323. Non-Viral, Lipid-Mediated DNA and mRNA Gene Therapy of the Central Nervous System (CNS): Chemical-Based Transfection
(1)
Department of Anesthesiology and Pain Medicine, Harborview Medical Center, University of Washington School of Medicine, Box 359724, 329 Ninth Ave., Seattle, WA 98104, USA
Abstract
Appropriate gene delivery systems are essential for successful gene therapy in clinical medicine. Cationic lipid-mediated delivery is an alternative to viral vector-mediated gene delivery. Lipid-mediated delivery of DNA or mRNA is usually more rapid than viral-mediated delivery, offers a larger payload, and has a nearly zero risk of incorporation. Lipid-mediated delivery of DNA or RNA is therefore preferable to viral DNA delivery in those clinical applications that do not require long-term expression for chronic conditions. Delivery of RNA may be preferable to non-viral DNA delivery in some clinical applications, because transit across the nuclear membrane is not necessary and onset of expression with RNA is therefore even faster than with DNA, although both are faster than most viral vectors. Here, we describe techniques for cationic lipid-mediated delivery of nucleic acids encoding reporter genes in a variety of cell lines. We describe optimized formulations and transfection procedures that we previously assessed by bioluminescence and flow cytometry. RNA transfection demonstrates increased efficiency relative to DNA transfection in non-dividing cells. Delivery of mRNA results in onset of expression within 1 h after transfection and a peak in expression 5–7 h after transfection. Duration of expression in eukaryotic cells after mRNA transcript delivery depends on multiple factors, including transcript stability, protein turnover, and cell type. Delivery of DNA results in onset of expression within 5 h after transfection, a peak in expression 24–48 h after transfection, and a return to baseline that can be as long as several weeks after transfection. In vitro results are consistent with our in vivo delivery results, techniques for which are described as well. RNA delivery is suitable for short-term transient gene expression due to its rapid onset, short duration of expression and greater efficiency, particularly in non-dividing cells, while the longer duration and the higher mean levels of expression per cell that are ultimately obtained following DNA delivery confirm a continuing role for DNA gene delivery in clinical applications that require longer term transient gene expression.
Key words
Non-viral Lipid-mediated Gene delivery Transfection RNA DNA siRNA Primary neuron s Post-mitotic Molecular therapy Transient CHO NIH3T3 1 Introduction
Gene therapy has the potential to significantly advance clinical medicine, but the risks and duration of gene delivery should be closely matched to the proposed clinical application [1]. Long-term expression after gene therapy is useful for diseases that require chronic levels of protein expression, such as inherited enzyme deficiencies, or cancer, and for these diseases viral vectors may offer advantages. For clinical applications in which only short-term gene expression is required or warranted, the delivery of nucleic acids (DNA or RNA , including mRNA, siRNA , shRNA, and microRNAs) by means of non-viral lipids provides a far more favorable risk/benefit ratio. Lipid-mediated transfection also offers other advantages over viral vectors, most notably safety, low immunogenicity, ease of preparation, and the ability to deliver payloads of nearly unlimited size [2, 3].
Cationic lipid-mediated gene transfer is particularly suited for transient gene expression, both in basic research and in selected clinical applications. Cationic lipids are commonly comprised of a polar headgroup and non-polar symmetric or dissymmetric carbon-based tail, and may also include Nuclear Localization Signals (NLS), antibodies, polymers, or other targeting moieties. Negatively charged nucleic acids condense and self-assemble into heterogeneous complexes when mixed with cationic lipids [4]. The structure and size of these complexes affect transfection efficacy and vary with temperature, concentration, charge ratio, buffer, time, and lipid composition. These lipid/nucleic acid complexes protect nucleic acids from degradation in the extracellular environment [5]. Numerous laboratories [6, 7], including our own [8], have investigated the limiting parameters of cationic lipid-mediated transfection with the goal of improving transfection efficiency [9]. Four general barriers to lipid-mediated DNA transfection include: (1) transport of the nucleic acid/lipid complex in the extracellular environment to target tissue(s); (2) association and uptake of the nucleic acid/lipid complex by the target cell [10]; (3) intracellular DNA or RNA release from the nucleic acid/lipid complex [11]; and (4) translocation of DNA to the nucleus [12], which is not required after RNA delivery. The primary barrier to DNA transfections in post-mitotic cells is assumed to be translocation of DNA to the nucleus [13]. While cationic lipid-mediated transfections work well with many types of cells [2, 3, 6], transfection of primary cell lines remained a problem [9, 14]. This transfection difficulty was generally attributed to markedly reduced or absent mitotic activity in primary cells, which are almost exclusively post-mitotic [14, 15]. In proliferating cells, nuclear translocation is mainly passive, and occurs during mitosis as the nuclear membrane breaks down [10, 16, 17]. Some nuclear translocation does still occur in non-proliferating cells, probably the result of passive movement through the nuclear pore complex (NPC) [18, 19].
Methods for avoiding the necessity of nuclear translocation of DNA have been reported, for example delivery of T7 promoter DNA plasmid systems to T7 polymerase-expressing cells [20], but these systems have limited clinical applications. Lipid-mediated RNA and DNA delivery to proliferating cells [21] as well as intramuscular injection of naked RNA and DNA have also been previously described [22]. Using RNA instead of DNA eliminates the necessity for nuclear translocation and thus has the potential to greatly improve transfection of post-mitotic cells.
We compared numerous lipids, cationic and otherwise, in a wide variety of in vitro and in vivo experiments, transfection of primary neuronal cells, delivery to proliferation-inhibited dividing cells [15], and in vivo imaging of reporter delivery and expression. Our studies show that mRNA and DNA are rapidly and robustly expressed, and offer safety advantages over viral vectors [15, 23]. RNA is 2–5 times more efficient at cell transfection than DNA based on the percentage of cells transfected. DNA delivery results in a higher level of expression under most cases, although with a longer time to expression, and a longer duration of expression.
This protocol is focused on DNA and mRNA delivery into primary neurons and in vivo. However, it is quite practical at first to work out the main parameters of liposomal formulation on fast growing, easily available cell types. That is why we include the experiments on CHO cells. The protocol includes the use of GFP and luciferase reporter transcripts. Both can serve as suitable reporter constructs and controls. Although the measurement of GFP expression by flow cytometry can be preferable for cell line transfection , flow analysis of neuronal cells is more difficult, and luciferase reporter is more suitable for analysis of neuronal transfection. In trouble shooting mRNA delivery, it is advisable to have DNA transfection controls in parallel.
We recommend the commercially available lipid TransFast™ Transfection Reagent (Promega, Madison, WI, USA) for RNA and DNA delivery. In our long experience Transfast is the closest in efficiency and low cytotoxicity to our novel lipid MLRI, which is available by special order but not commercially (see Note 1 ). TransFast™ Transfection Reagent is comprised of the synthetic cationic lipid (+)-N,N{bis (2-hydroxyehyl)}-N-methyl-N-{2,3-di(tetradecanolyloxy)propyl} ammonium iodide and the neutral lipid, DOPE (Promega). Transfast is supplied by Promega as a lipid film in glass vials under argon gas that must be rehydrated with DDW (nuclease- and RNase-free). Liposome reagents specifically designed for transfection applications often incorporate synthetic cationic lipids, such as the neutral lipid DOPE or cholesterol, to improve efficacy. DOPE has been demonstrated to enhance the gene transfer ability of certain cationic lipids [24]. We optimized transfection formulations of GFP encoding RNA and DNA vectors using flow cytometry, by varying charge ratio, formulation time, concentration, and temperature, to obtain the maximum percentage of GFP-expressing cells. Ideally, each cell line of interest should have optimal formulations confirmed before proceeding to in vivo experiments.
2 Materials
2.1 Cells
1.
CHO cells (ATCC, Rockville, MD, USA).
2.
NIH3T3 cells (ATCC, Rockville, MD, USA).
3.
Primary neuron al cells dissected from the cortex of day 17 Sprague–Dawley rat fetuses.
2.2 Vectors (See Note 2 )
1.
β-Globin luciferase DNA vector (βglucβg). This vector (gift of Jon Wolf, MD, University of Wisconsin) [21, 22], which contains β-globin stabilizing elements, was used for time course experiments in primary neuronal cells. β-Globin is one of the most stable naturally occurring mRNAs found in nature, and the stabilizing elements from β-globin mRNA have been used in in vitro mRNA transcripts to design long-lived mRNA. To produce the β-globin stabilized transcripts, cloning procedures were carried out essentially as described in Molecular Cloning : A Laboratory Manual [25]. Xenopus laevis β-globin sequences were derived from the plasmid pSP64 T [26], with the 5′ β-globin sequences obtained as the HindIII/Bgl II fragment and the 3′ β-globin sequences released as the Bgl II/EcoRI fragment. These 3′ sequences include a terminal polynucleotide tract of A23C30. T7 RNA polymerase transcription templates, as well as various mRNAs produced from them, are outlined in [21]. We did not see significant differences in stability between the various mRNAs we produced and analyzed.
2.
Luciferase DNA Vector (pNDlux.2). The pND luciferase expression vector (gift of Gary Rhodes, PhD, University of California-Davis) contains the CMV immediate early promoter (HCMV IE1) and CMV IE1 intron, a polylinker cloning site, and the RNA terminator/polyadenylation site from bovine growth hormone (BGH). These elements are contained in a pUC19 replicon. cDNA encoding the luciferase gene from the firefly Photinus pyralis (pGL2+, Promega, Madison, WI) was cloned into expression vector pND to give the plasmid pNDlux.2.
3.
GFP DNA Vector (pNDeGFP). Full-length enhanced GFP (eGFP, a variant of jellyfish Aequorea victoria GFP) cDNA (pEGFP, Clontech, Palo Alto, CA) was subcloned into the pND vector described above to give the DNA vector, pNDeGFP (also a gift of Gary Rhodes).
2.3 Vectors for mRNA Transcripts (See Note 3 )
1.
β-Globin Vector for GFP mRNA Transcript (see Note 4 ): Briefly, eGFP was subcloned into the β-globin vector using Nco1 and Xba1 restriction enzymes to form β-globin-enhanced Green Fluorescent Protein (β-globin eGFP) vector. β-Globin eGFP was linearized with restriction enzyme Dra I after elimination of RNases by proteinase K treatment and phenol/chloroform extraction. After precipitation in ethanol mRNA transcripts encoding the reporter gene sequence for firefly luciferase were transcribed in vitro using both mMessage mMachine transcription kit and the Ultra mMessage mMachine transcription kit (Ambion, Austin, TX). The mMessage mMachine and Ultra mMessage Machine kits are identical in content except for the mRNA cap structure included. mMessage mMachine kits contain the “standard” mRNA cap while the Ultra kit contains the Anti Reverse Cap Analog (ARCA).
2.
β-Globin Vector for Luciferase mRNA Transcript (see Note 4 ): Luciferase-expressing mRNA was also produced using the pT7βglucβg plasmid (gift from Jon Wolff, M.D., University of Wisconsin). Procedures similar to item 1 above were followed.
3.
pT7OmegaGFPA Vector for GFP mRNA Transcript: The plasmid pT7OmegaGFPA50 (gift from Robert Malone, University of California-Davis) was also used as a template for GFP expression. The GFP-expressing mRNA contains the Omega 5′ UTR and 3′ poly-adenylated tail from Tobacco Mosaic Virus (TMV). 5′ and 3′ UTR from TMV have also been shown to improve intracellular mRNA stability and lead to translational enhancements in eukaryotic as well as prokaryotic cells, much like the β-globin UTR described above [27–29]. Transfections in our laboratory comparing time course of expression between luciferase vectors containing TMV UTR and β-globin UTR vectors revealed nearly identical kinetics and levels of expression (data not shown).
2.4 Equipment
1.
5 % CO2 incubator at 37 °C.
2.
Low speed centrifuge.
3.
Dual channel FACScan or multi-channel Caliber flow cytometer (Becton Dickinson, San Jose, CA, USA) equipped with a single 488 nm argon laser (GFP fluorescence measured with a 530 nm band pass filter and Tri-Color fluorescence measured with a 675 band pass filter).
4.
CellQuest (Becton Dickinson, East Rutherford, NJ, USA) or equivalent flow cytometry analysis software.
5.
Monolight 2010 (Becton Dickinson, East Rutherford, NJ, USA) or equivalent.
6.
Stereotaxic small animal surgery frame (Stoelting, Wood Dale, IL, USA).
7.
Syringe infusion pump (Stoelting, Wood Dale, IL, USA).
2.5 Supplies
1.
75 cm2 cell culture flasks.
2.
60 mm Petri dishes.
3.
15 ml sterile tube.
4.
Pasteur pipette tubes.
5.
40 μm nylon nets.
6.
Intraventricular canula.
7.
Microscope slides (Columbia Diagnostics, Inc., Springfield, VA, USA) poly-l-lysine-coated and air-dried for a minimum of 2 h.
2.6 Media
1.
HAMS F12 media (Life Technologies, Gaithersburg, MD, USA).
2.
DMEM media.
3.
OptiMem media.
4.
10 % bovine calf serum.
5.
PBS.
6.
Ice-cold sterile PBS.
7.
Neural Basal Medium (NBM), containing 0.5 mM l-glutamine, 1× B27, 50 ng/ml neural growth factor , supplemented with 0.25 % trypsin-EDTA and 20 μl DNase I.
2.7 Templates for RNA Synthesis
1.
eGFP and luciferase mRNA transcripts as controls for optimization (β-globin eGFP is shown in Subheading 3) (see Note 5 ).
2.
mRNA or short RNAs (siRNA , hRNA, microRNAs) of your desired sequences using standard techniques (in vitro transcription and purification using RNAse-free laboratory techniques, as in Red Book or other standard lab reference) (see Note 6 ).
2.8 Nucleic Acid Delivery
1.
Restriction enzymes (we used Nco1, Nde1, Dra I, and Xba1).
2.
Proteinase K.
3.
Phenol/chloroform.
4.
Ethanol.
5.
T7 mMessage mMachine transcription kit (Ambion, Austin, TX, USA).
6.
Enhanced Luciferase assay Kit (BD Bioscience, East Rutherford, NJ, USA).
7.
TransFast™ Transfection Reagent (Promega, Madison, WI, USA).
8.
Neutral lipid DOPE (Promega, Madison, WI, USA) or cholesterol.
9.
eGFP or luciferase pDNA control vectors (Clontech, Mountain View, CA, USA).
10.
Trypsin.
11.
Dulbecco’s phosphate buffered saline with Ca2+ (DPBS).
12.
Annexin V conjugated with biotin (CalTag, Burlingame, CA, USA) in DPBS.
13.
Streptavidin conjugated with a Tri-Color fluorophore (CalTag, Burlingame, CA, USA) in DPBS.
14.
Lysis buffer.
15.
CSF (cerebral spinal fluid).
2.9 Tissue Preparation for Reporter Protein Localization After In Vivo DNA or mRNA Delivery
1.
Iced saline for perfusion.
2.
Iced 4 % paraformaldehyde in 0.1 M, pH 7.4 sodium phosphate-buffered saline (PBS).
3.
Paraformaldehyde fixative at 4 °C.
4.
PBS containing 20 % glycerol at 4 °C.
2.10 Diaminobenzidine Immunohistochemistry
1.
Diaminobenzidine (DAB).
2.
24-well plates for free-floating sections.
3.
ExtrAvidin® peroxidase system (Sigma, St. Louis, MO, USA).
4.
Poly-l-lysine-coated microscope slides for slide-mounted sections (Columbia Diagnostics, Inc., Springfield, VA, USA).
5.
0.1 % Hydrogen peroxide (H2O2).
6.
4 % paraformaldehyde (described above).
7.
Blocking buffer: 0.3 % Triton X-100, 3 % bovine serum albumin (BSA), 10 % normal goat serum (NGS), Ca2+ and Mg2+-free PBS.
8.
Biotin-conjugated secondary Ab (biotinylated goat anti-mouse; Vector Labs, Burlingame, CA, USA), tertiary horseradish peroxidase-conjugated probe (ExtrAvidin® peroxidase system).
9.
50 mM Tris–HCl at pH 7.6.
10.
0.5 mg/ml 3,39-DAB with 0.03 % H2O2 as the peroxidase substrate.
11.
Mouse monoclonal neuron-specific nuclear protein anti-NeuN (1:50; EMD Millipore, Billerica, MA, USA).
12.
Rabbit polyclonal antiluciferase antibody.
2.11 Documentation of Results
1.
Nikon 600 microscope with camera mount (Nikon Instruments, Melville, NY, USA) or equivalent.
2.
Photoshop and Polaroid SprintScan slide scanner for film negatives or slides.
3.
Fuji Pictrography 3000 (Fuji Photo Film, Elmsford, NY, USA), or equivalent.
4.
Nikon Eclipse TS100 Inverted microscope (Nikon Instruments, Melville, NY, USA) or equivalent.
5.
High-resolution digital camera (SPOT; Diagnostic Instruments, Sterling Heights, MI, USA) or equivalent.
3 Methods
3.1 CHO Cells
1.
Culture CHO cells in 75 cm2 cell culture flasks with HAMS F12 media at 37 ° C in a 5 % CO2 environment (see Note 7 ).
2.
Split cells 48 h prior to transfection .
3.
Plate at 60 % confluence.
3.2 Primary Neuronal Cells
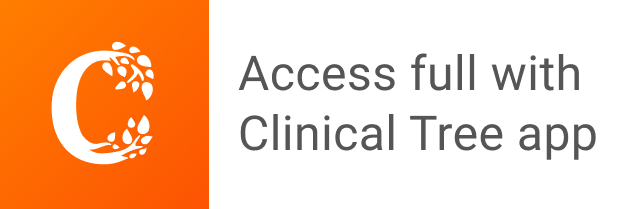