Introduction
During the first decade of the 21st century, many major advances have revolutionized neurocritical care. These changes have been achieved mainly because neurocritical care now is regarded as a distinct specialty in its own right, necessitating specialists familiar with patients with neurologic injury. Application of new technologies toward the central nervous system (CNS) in an effort to understand and manage the physiology of the brain has been central to this mission. Such technologies include intracranial pressure monitoring, brain oxygen and cerebral blood flow (CBF) monitoring, microdialysis techniques, and jugular venous blood saturation monitoring, to name a few. Each of these technologies used in neurocritical care, however, have important limitations. First, their use is generally limited to major medical centers in part because of the personnel required to install and manage these devices, the training required, and the cost of implementation. Second, some of devices are frequently criticized as being too global and therefore nonspecific in their measurements, or conversely too focal and then not representative of the entire neurologic system. Third and perhaps most important, most of the devices and in particular those to measure the gold standard parameter, intracranial pressure (ICP), are invasive and so there is a reluctance to insert these devices. It seems that technologies able to take neurocritical care to the next level will need to be noninvasive to garner widespread use, as well as multimodal, because clinical experience has indicated that the neurologic system cannot be optimally managed without an integrated approach that factors the multiple important determinants of brain pathophysiology.
Because of its anatomy, the brain is not an easy organ on which to perform noninvasive monitoring. The surrounding skull represents an important barrier to ultrasonic insonation, for example. Thanks to technologic refinements, various direct sensors used in neurocritical care have become safer and less invasive. They have been miniaturized, sometimes made wireless, and the infection risk has decreased as these monitors are excluded from intracranial contents such as the cerebrospinal fluid (CSF). In the majority of acute neurologic injuries the small risk of sensor placement is outweighed by the clinical benefits of having better insight into assessment of the timing and extent of secondary insults such as ischemia. Nevertheless, the benefits of noninvasive brain monitoring are obvious, particularly in cases with known contraindications (e.g., hepatic encephalopathy in which an underlying coagulopathy increases the risk of hemorrhage from insertion of an invasive monitoring device) or when the potential benefits of invasive monitoring are unclear (e.g., moderate stroke, moderate and mild head injury, or eclampsia, among others).
This chapter reviews some of the noninvasive technologies available now as well as on the horizon that may enhance neurocritical care.
Noninvasive Intracranial Pressure and Cerebral Perfusion Pressure Monitoring
ICP can be estimated noninvasively using various methods: (1) tympanic membrane displacement, (2) fontanometry, (3) analysis of transcranial Doppler (TCD) pulse waveform, or (4) ocular nerve sonography. However, none of these noninvasive methods can be considered reliable or precise enough to be used routinely in the clinical management of intracranial hypertension. Nor is there a validated noninvasive system that can replace invasive ICP monitors for quantification of ICP. Instead current noninvasive monitors provide supporting qualitative data that may be helpful in making decisions about use of more invasive direct measurement devices.
Various methods of noninvasive ICP measurement are described in the contemporary literature :
- ▪
Prediction of ICP from noninvasive transocular venous and arterial hemodynamic measurements (reported 95% confidence interval for prediction ±12 mm Hg)
- ▪
Change in a skull diameter (no consistent 95% percent limit)
- ▪
Change in blood flow velocity in the straight sinus (estimated 95% confidence limit is low, ±8.8 mm Hg, but this is a difficult measurement to make in adults)
- ▪
Methods based on electroencephalography (reported lowest 95% limits for prediction ±8 mm Hg)
- ▪
Methods based on magnetic resonance imaging (MRI) analysis of CSF volume, as well as arterial blood inflow and venous blood outflow from the cranium (there is no consistent 95% confidence limit reported)
- ▪
MRI or ultrasound-based measurement of the optic nerve sheath diameter (95% confidence limit ±20 mm Hg)
Intracranial Pressure Monitoring Based on Transcranial Doppler
Methods using TCD ultrasonography are discussed in more detail because this technology is commonly used to noninvasively assess ICP. Aaslid’s design of TCD sonography in 1982 permitted bedside monitoring of CBF velocity (the instantaneous speed of flowing blood, typically in units of cm/second), which may be used assuming vasospasm is absent as an index of the CBF (the instantaneous volume of flowing blood, typically in units of mL/second; see also Chapter 30 ). The measurement may be conducted noninvasively, repeatedly, and even continuously. This is a “big tube technique,” which measures arterial blood flow velocity in the major branches of the circle of Willis, most commonly the middle cerebral artery (MCA). A compliant branch of the MCA may be interpreted as an implanted pressure transducer, in that the pattern of blood flow within the MCA is modulated by transmural pressure (i.e., cerebral perfusion pressure [CPP]) and the distal vascular resistance (also modulated by CPP), and therefore analysis of MCA blood flow should produce a measure of the net pressure acting on the “transducer” (again, the MCA). But the calibration factor for the MCA, the nonlinear distortion, and other factors (in particular, their stability in time) are unknown.
There is a reasonable correlation between the pulsatility index (PI) of the MCA blood flow velocity and CPP after head injury, but absolute measurements of CPP cannot be extrapolated. Others suggest that critical closing pressure (CCP), derived from flow velocity and arterial pressure waveform, approximates ICP. The accuracy of this method has, however, never been satisfactorily demonstrated. Aaslid et al. proposed that an index of CPP could be derived from the ratio of the amplitudes of the first harmonics of the arterial blood pressure and the MCA velocity (detected by TCD sonography) multiplied by the mean flow velocity in the MCA:
nCPP = A1 / F1 × FVm
where F1 and A1 are first harmonic components of flow velocity and arterial pressure pulse waveforms, and FVm is time-averaged flow velocity. However, the 95% confidence limit for predicted values is as wide as plus or minus 20 mm Hg.
CPP affects the shape of the blood flow velocity waveform. However, arterial pulse waveform, heart rate, tension of arterial carbon dioxide (CO 2 ), distal vascular resistance, and even age, all affect the flow velocity (FV) waveform as well. Some simple formulas to assess CPP noninvasively from arterial blood pressure (ABP) and FV waveforms have been proposed. One particular calculation has achieved satisfactory accuracy (error less than ±10 mm Hg in more than 80% measurements ; including in traumatic brain injury [TBI] patients, shown in work by Mourad, Kliot et al.):
nCPP = MAP × FVd / FVm + 14
where FVd is diastolic flow velocity; FVm is mean flow velocity, and 14 mm Hg is “calibrating constant” for TBI patients.
nCPP is useful both to estimate absolute CPP and to monitor changes in CPP in time ( Fig. 46.1 ). The 95% confidence limit for estimation of CPP is 12 mm Hg (prospective trial) and 18 mm Hg in retrospective analysis of these authors’ own database. Although this seems to be satisfactory for CPP, such precision is not good enough to estimate ICP.

The pulsatility index (measured by TCD) increases as ICP increases. The prediction of absolute ICP using PI may not to be accurate enough because many other factors can influence PI such as arterial pulse, heart rate, PaCO 2 , vascular tone, proximal stenosis, and spasm, among others. However, data from Bellner et al. suggest a very narrow 95% confidence limit for prediction in head-injured patients: ±4.2 mm Hg. The same prediction limit derived from data obtained at Cambridge and the University of Washington is plus or minus 20 mm Hg. Such a huge discrepancy ought to be explained.
A moving-average model of transmission between ABP and ICP, modified by the relationship between ABP and FV gives a mean absolute error of 6 mm Hg and 95% confidence limit for predictor of plus or minus 16 mm Hg. The method is based on analysis of a large database of patients with homogeneous pathology who received ICP, ABP, and FV direct monitoring and is most probably pathology dependent. The method is useful for monitoring ICP changes over time ( Fig. 46.2 ). However, a change in PaCO 2 , vessel spasm and proximal stenosis can confound the results.

TCD methods (with easy access to bilateral Doppler machines) theoretically allow the assessment of interhemispheric gradients of ICP or CPP. As long as CSF communicates freely between different fluid cavities within the brain, there should not be any substantial differences in regionally measured ICP. Direct measurements of pressures in two CSF compartments are performed rarely. In head injury, intrahemispheric pressure gradients are reported with interhemispheric ICP differences greater than 20 mm Hg measured in one severely injured patient. Other studies of patients with focal injuries or intracranial masses show a median interhemispheric difference of 5.5 mm Hg after craniotomy but before parenchymal resection, ranging from 3 to 16 mm Hg. There are intrahemispheric pressure gradients in critical closing pressure and noninvasive CPP associated with midline shift, side of contusion (assessed using computed tomography [CT]), or side of craniectomy. Both nCPP and CCP measurements indicate that cerebral perfusion or level of cerebrovascular dilation is greater on the side of a contusion or more swollen brain in the case of midline shift. This may support the hypothesis that vascular expansion and not a brain tissue volume increase is associated with side-to-side differences seen on CT scan. This hypothesis may be further supported by the observation that cerebral autoregulation is worse on the side of contusion or brain expansion.
Intracranial Pressure Monitors Based on the Optic Nerve
The optic nerve sheath (ONS) is an extension of the dura mater, and the space within the sheath is continuous with the intracranial contents. Therefore pressure in the ONS increases when ICP increases. This can be detected by physical examination (i.e., funduscopy) to detect papilledema. The Frisen scale can be used to grade the severity of papilledema (0, normal, through 5, severe). Although papilledema indicates increased ICP, funduscopy is not suited to monitoring patients in the NCCU. First, it is a qualitative “point-in-time” assessment. Second, the sensitivity and specificity to detect papilledema vary among medical specialists and may depend on pupillary dilation. Third, in acute brain injury, papilledema may take several hours to develop after ICP increases. Fourth, the absence of papilledema does not preclude increased ICP. In addition, spontaneous venous pulsations, the absence of which are consistent with increased ICP, are absent in about 10% of normal subjects. Fifth, the optic nerve does not swell in atrophic areas (i.e., papilledema is not reliable in patients who have had previous optic nerve injury). To overcome the limitations of funduscopy, several techniques based on evaluation of the ONS have been used to evaluate ICP, including (1) ophthalmodynamometry, (2) scanning laser tomography (SLT), (3) optical coherence tomography, and (4) ONS ultrasound. In addition, color Doppler ultrasonography to insonate the ophthalmic artery and the central retinal artery and vein can be used. Although these techniques can be used to quantify ICP, none is suitable for ICP monitoring in the ICU at present.
Ophthalmodynamometry
This technique is an attempt to quantify ICP. First, the baseline intraocular pressure (IOP) is measured. Then a suction cup is placed on the eye, and IOP increased until the central retinal vein collapses. This pressure added to the baseline IOP indicates the central retinal vein venous outflow pressure that has a linear relationship with ICP.
Scanning Laser Tomography
SLT uses a laser to scan the retinal surface. It is primarily used in patients with glaucoma but has been used to quantify papilledema in patients with idiopathic intracranial hypertension in which optic nerve height and volume measurements are associated with opening pressure on lumbar puncture.
Optical Coherence Tomography
Infrared light to measure total retinal thickness, retinal nerve fiber layer thickness, and optic nerve head morphology is used to quantify papilledema. This technique can be used to distinguish normal nerves from mild papilledema but at present is not suited to NCCU monitoring.
Optic Nerve Sheath Ultrasound
Measurement of optic nerve diameter using ultrasound, typically using a frequency between 5 and 10.5 MHz, is the most studied noninvasive method of ICP assessment based on the ONS. The technique is relatively easy to learn and interobserver variation is low. The ONS begins to expand when the ICP is greater than 15 mm Hg, and beyond this level is a linear correlation between ONS enlargement and ICP increases. An ONS diameter on ultrasound between 4.8 and 5.9 mm measured 3 mm posterior to the globe is considered the threshold value to identify ICP greater than 20 mm Hg. Although this technique has promise, it has not been used to monitor ICP in the ICU because eye injury or swelling may limit its use, off-axis measurement of the ONS diameter provides an incorrect value, and artifacts may limit quality.
Continuous Monitoring of Cerebral Autoregulation
Cerebral autoregulation is an essential intrinsic, self-protecting brain mechanism in many disorders that require NCCU care (e.g., TBI, subarachnoid hemorrhage [SAH], and stroke). On the other hand some therapies for severe TBI, in particular the Lund concept, are based on the premise that autoregulation is impaired. Methods for noninvasive assessment of autoregulation through study of CBF can be divided into (1) tests in which an external stimulus to challenge regulation mechanisms is applied or (2) methods suitable for continuous monitoring, that is, without an external stimulus. Methods to examine autoregulation include (1) static rate of autoregulation, (2) lower body negative pressure, (3) tilt test, (4) CO 2 reactivity, (5) leg-cuff test, and (6) transient hyperemic response ( Fig. 46.3 ). These tests provide information at a point in time, but in many conditions, cerebral autoregulation may fluctuate quite rapidly, particularly in NCCU patients. In such cases, continuous monitoring of autoregulation is clinically useful. These methods rely on analysis of spontaneous continuous fluctuations of MAP or CPP. Thanks to refinement of computer-assisted methodology, fluctuations of MAP greater than 5 mm Hg are suitable to retrieve information about autoregulation. In some cases information is less precise than in tests using an external stimulus, but the ability to time-average continuously obtained autoregulation indices can improve the signal-to-noise ratio of measures of autoregulation.

System Analysis: Phase Shift and Transfer Function Between Arterial Pressure and Flow Velocity Waves
In this methodology it is presumed that autoregulation mechanisms can be described by a linear dynamic system with arterial blood pressure as input and blood flow velocity the output. The fast pulsatile component associated with the cardiac cycle is probably too fast to help assess autoregulation, in part because cerebral autoregulation acts primarily through altering the diameter of the secondary cerebral arterioles on time scales of a few seconds in healthy individuals, and longer for injured patients. However, respiratory waves (from 0.4-0.16 Hz) and slow waves (0.05-0.005 Hz) are slower and therefore they can carry information about cerebral autoregulation. The first step in processing FV and ABP time-series is therefore filtering out pulsatile components, typically with a low pass filter of cutoff frequency of 0.5 Hz. A noninvasive method to derive autoregulatory status from natural fluctuations in MCA flow velocity and ABP involves the assessment of phase shift between the superimposed respiratory or slow ABP and blood FV waves during spontaneous recordings or slow breathing. Coherence function, which is a Fourier transform of normalized cross-correlation between ABP and FV series is calculated. The modulus of coherence indicates how strong components of given frequency are associated with each other. Another transformation method is a transfer function between ABP and FV. It is a complex function, having its gain and phase shift. Phase indicates which variable changes before another. A zero-degree phase shift (e.g., both ABP and FV change at the same time, without delay) indicates absent autoregulation, whereas a positive phase shift (>30 degrees, i.e., FV changes before ABP) indicates intact autoregulation ( Fig. 46.4 ). Higher FV (output) and ABP (input) transfer function also indicate worse autoregulation. In both methods, the absolute value of coherence between FV and MAP should be more than 0.5 in the analyzed frequency bandwidth. Both methods presume a linear relationship between changes in FV and MAP within a studied range of fluctuations.
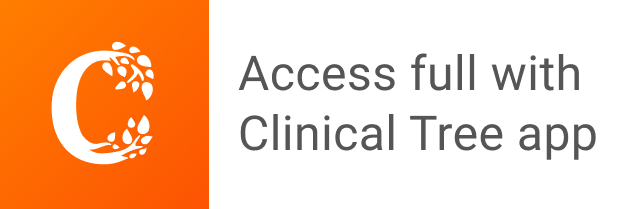