Nonresective and Neuromodulatory Treatments of Refractory Epilepsy As many as 10 to 40% of pediatric patients with epilepsy have intractable seizures, despite the use of multiple antiepileptic agents.1–3 Seizure control is especially important in the pediatric population because persistent seizures can profoundly impair cognitive and psychosocial development.4,5 In these patients, surgical intervention can be offered as an alternative means of seizure control. Epilepsy is a diverse entity caused by a range of distinct pathologies. For this reason, multiple surgical interventions have emerged for the treatment of various disease processes. Beginning with Horsley’s excision of neocortical tissue for focal motor seizures in 18866 and the pioneering work of Foerster, Penfield, Jasper, and others, epilepsy surgeries have classically been resection procedures with the goal of removing or ablating epileptogenic tissue. These procedures include both focal resection (lesionectomy) when discrete anatomical lesions like neoplasms and vascular anomalies can be identified, and wider excisions (corticectomy, lobectomy, hemispherectomy) when the underlying pathology is more diffuse, as in malformations of cortical development and mesial temporal lobe sclerosis. The goal of resection procedures is curative, with the hope that removal of a presumed primary epileptogenic focus will prevent further seizures. These strategies of focal resection and their application in children are discussed in other chapters. In many patients, resection is not a viable option, either because there is no clearly identifiable seizure focus, there are multiple diffuse epileptogenic foci, or an epileptogenic focus exists in nonresectable eloquent cortex. In such cases, nonresective interventions should be considered. Instead of the resection of epileptogenic tissue, the goal of these interventions is to modulate the existing circuits to control seizure initiation, propagation, and/or generalization. Nonresective treatments are generally palliative rather than curative, with the intended goal of reducing the frequency or severity of seizures. Two subtypes of procedures fall into this category of neuromodulation: disconnection procedures and neurostimulation. Disconnection procedures transect afferent and efferent fibers in an attempt to functionally isolate surrounding epileptogenic tissue—either directly or indirectly interrupting pathways that mediate excitation and seizure propagation from a primary focus, or disrupting more complex dynamics, such as subcortically mediated synchronization or network-level coupling, to prevent the generalization of pathologic synchronous neural activity. The mechanism of action of neurostimulation procedures is more complex. Generally speaking, an electrical stimulus is initiated to “modulate,” either directly or indirectly, through the excitation of excitatory or inhibitory pathways of the neural networks involved in epileptogenesis. We review here the most common nonresective neurosurgical strategies for intractable epilepsy, with a particular focus on corpus callosotomy and vagus nerve stimulation. Multiple subpial transections (MSTs) were introduced by Morrell and colleagues for medically refractory partial epilepsy originating in eloquent regions of neocortex.7 The rationale for MSTs is based on the concept that normal neurophysiologic function relies primarily on vertically oriented fibers, independently of the lateral propagation of seizure activity. Transecting the cortex in the horizontal plane disrupts seizure spread while sparing normal function. Multiple retrospective studies have demonstrated that MSTs are safe and efficacious8–13; however, this has not yet been validated by a prospective trial. To create MSTs, a fine microdissection device is introduced through the pia and is used to make vertical transections oriented perpendicularly to the long axis of a gyrus, with parallel transections repeated at approximately 5-mm intervals across the epileptogenic region. Postsurgical results reveal a generally excellent seizure response (60 to 70% patients achieved > 95% reduction in seizure frequency in a recent international meta-analysis); the comparable responses for MSTs alone and the combination of MSTs plus resection in partial seizures validate the disconnection paradigm as a viable means of seizure control.14 Functional hemispherectomy (hemispherotomy) is analogous to MSTs in that it attempts to circumscribe and isolate an epileptogenic focus; however, the target region is an entire hemisphere rather than a single lesion, and the isolated neural circuits are completely disconnected from the rest of the brain. This procedure and several variants are modifications of the original hemispherectomy procedure,15 with the frontal and occipital poles left intact but disconnected to avoid the superficial cerebral hemosiderosis related to hemorrhage into the extended resection cavity.16 Functional hemispherectomy, which is discussed in Chapter ▶ 75, is different from the other disconnection procedures considered here in that there is no intention of preserving the function of the neurons left behind but disconnected. Division of the corpus callosum to prevent seizure generalization is the other major type of neurosurgical disconnection procedure. Corpus callosotomy has been shown to be safe and effective, with the greatest benefit for patients with intractable drop attacks and/or generalized tonic–clonic seizures.17–19 Dandy initially reported the feasibility of operative corpus callosum division after using this maneuver for pineal tumor resections.20 Disconnection of the cerebral hemispheres to treat intractable seizures was then proposed by Van Wagenen and Herren soon thereafter,21 contemporaneously with pioneering work by Erickson on the effect of callosotomy on seizure propagation in the monkey.22 Although additional midline structures, including the interhemispheric commissures, massa intermedia, and fornix, have been the targets of disconnection procedures, there is no clear benefit beyond that of corpus callosotomy alone. Today, understanding is evolving about both the functional implications of corpus callosum division and the possible mechanisms of seizure control with corpus callosotomy. The corpus callosum is the largest commissural connection in the human brain, consisting of an estimated 200 million interhemispheric fibers between primarily homologous areas of neocortex.23 Most callosal fibers connect higher-order association areas, with only minimal callosal projections observed between primary motor or sensory cortices. Although the anatomical organization of the callosal fiber tracts is now well-established, the mechanisms mediating the transfer of information are still a topic of debate.24 Many studies support an excitatory role, in which the corpus callosum allows the interhemispheric integration of information. Others suggest that callosal fibers impose an inhibitory effect on the opposite hemisphere, leading to increased lateralization and allowing more efficient processing.25,26 Anatomically, the corpus callosum demonstrates a heterogeneity of fiber composition and connectivity that underlies its various physiologic functions. Despite their relatively sparse representation in the callosum, connections between primary and secondary sensorimotor regions comprise the majority of large-diameter myelinated callosal fibers in the primate brain.23,27 This distribution of fast-conducting fibers is consistent with hypotheses that fast interhemispheric processing via the corpus callosum mediates certain midline fusion tasks, such as central vision, depth perception, binaural sound localization, and aspects of bimanual coordination.28–31 In contrast, interhemispheric connections between higher-order neocortical regions, such as the prefrontal cortex and extrastriate visual cortex, consist primarily of small-diameter unmyelinated fibers. Such slow-conducting fibers have been theorized to mediate the integration of more processed information between associative cortices.27 There is also a generally rostral-to-caudal topography of callosal fibers corresponding roughly to the neocortical regions they interconnect: prefrontal fibers in the rostrum and genu; motor, somatosensory, superior temporal, and parietal fibers in the body; and inferior temporal and visual fibers in the splenium.32,33 Neurotracing studies suggest that the vast majority of these interhemispheric connections are homotopic, but heterotopic callosal fibers mediating interhemispheric parallel processing between different associative areas may also play a role.34 Likely the addition of tractography with diffusion tensor imaging and improved functional imaging and electrophysiologic modalities will help to elucidate the functional aspects, circuitry, and networks delivered in communication through the corpus callosum. Various animal and human models have been studied to better elucidate the function of the corpus callosum. Experiments in callosotomized animals first established a role for the corpus callosum in the somatosensory transmission of information between the hemispheres.35 However, its involvement in more complex cognitive function required direct evaluation in human models and human pathologic conditions. Congenital callosal agenesis has been extensively studied and has contributed to our current understanding of callosal function.36,37 However, these studies should be interpreted with caution because patients with congenital callosal agenesis often have other structural abnormalities (e.g., absence of the anterior commissure and hippocampal commissure).38 Furthermore, with congenital absence of the callosum, reorganization and functional compensation likely occur, given the neural plasticity of the developing brain.39–41 In contrast, the ability of the brain to compensate for lesions acquired postnatally is more limited. The most meaningful functional data have been generated in human patients with a postsurgical split brain. Over the past 40 years, a growing neuropsychological literature has accumulated describing the effects of partial or complete callosotomy in patients with intractable epilepsy. In the cognitive nomenclature, the condition resulting from the callosotomy was termed split brain.42–44 The varying deficits and degrees of impairment seen after callosotomy indicate the subtle and diverse nature of callosal function. A characteristic acute neurologic syndrome occurs in the first few postoperative days and includes most frequently mutism as well as nondominant arm and leg apraxia, bilateral Babinski signs, and urinary incontinence.45,46 These complications are almost always transient (although left-sided apraxia can persist for weeks or in rare cases be permanent) and may result from intraoperative retraction and manipulation rather than callosal division. Most of the persisting deficits following callosotomy involve the incomplete integration of information processing across hemispheres, often referred to in aggregate as the disconnection syndrome.47 In general, these deficits are subtle, and controlled neuropsychological testing is often required to elicit them. There is limited interhemispheric transfer of unilaterally presented perceptual information, such as visual and shape information. After callosotomy, patients also show deficits in the temporal coupling of continuous (but not discrete) bimanual movements,29 as well as difficulty in learning new tasks requiring bimanual cooperation.48 However, previously learned bimanual tasks, such as playing the piano and tying shoelaces, are preserved, suggesting a callosal role in the initial integration of complex motor tasks before eventual transfer to subcortical and cerebellar structures. This is consistent with human studies showing interregional and interhemispheric coherence of electroencephalographic (EEG) signals across premotor and sensorimotor regions during the acquisition of bimanual tasks.49 Language processing also exhibits deficits after callosotomy, which follows from the strong tendency of the human brain for lateralized language dominance. Images projected to the language-dominant left hemisphere (right visual field) can be easily described but cannot be verbalized when they are projected to the nondominant hemisphere despite their accurate reporting via nonverbal means (pointing). Although many models support a transcallosal excitatory integration of information, there is evidence that some callosal function may be inhibitory in nature. Activation of the motor cortex by a conditioning stimulus or direct electrical or transcranial magnetic stimulation causes inhibition of the contralateral motor cortex in animals and humans, likely via a transcallosally mediated activation of local inhibitory interneurons.50–52 After callosotomy, the normally unitary attention system can function as individual attentional processes,53,54 indicating a crucial role for the callosum in generating an integrated visuospatial attention system. Dual attentional processes may be prevented by interhemispheric inhibition in the normal brain, and the corpus callosum may have an integral role in generating selective attention. Tonic inhibitory processes are important to consider because disconnection procedures can produce a deafferentation of external inhibition to a region, which is a possible mechanism behind the occurrence of postoperative seizures. Perhaps the most dramatic complication of callosotomy is the alien hand syndrome, which represents a disruption of perceived conscious will and volition.55 Originally described by Goldstein in 1908 and further characterized by Akelaitis in 1945, patients with alien hand syndrome experience the subjective feeling of an upper limb performing complex motor acts outside their own volitional control.56 In addition to the striking intermanual conflict that most vividly demonstrates the syndrome, other signs of alien hand syndrome include automatic mirror movements of the unaffected hand, enabling synkinesis, grasp reflex, magnetic apraxia or impulsive groping, and utilization behavior. Alien hand syndrome can be divided into cases arising from callosal pathology or unilateral lesions of the cortex, in which the “alien” hand is always contralateral to the lesion. In the “callosal” subtype, alien hand syndrome results from surgical transection, each with a signature symptomatology that results from damage to distinct anatomical structures.57 The subtype of “callosal” alien hand syndrome results from surgical transection of the anterior corpus callosum or ischemia or rupture in the anterior cerebral artery circulation, presumably affecting fibers between premotor and motor areas. This subtype typically manifests as disrupted volitional control of the nondominant left hand. Although a relatively rare complication of callosotomy, the alien hand syndrome suggests that the corpus callosum participates in the construction of a unitary perception of “conscious will” by integrating either the performance of volitional action or the subjective perception of such behavior across hemispheres. The goal of corpus callosotomy is the palliative treatment of intractable generalized seizures, but the physiologic mechanism of seizure control produced by the division of callosal fibers remains unclear. The initial rationale behind callosotomy for seizure control was that the interruption of transcallosal pathways would prevent interhemispheric synaptic activation and seizure propagation. However, more recent evidence suggests that the callosal role in epileptogenesis may be more complex. In some studies of EEG changes after callosotomy, preoperative bilaterally synchronous epileptiform discharges are transformed into primarily lateralized or asynchronous ones, suggesting a callosally mediated propagation of seizure activity from one hemisphere to another.58–60 However, other evidence supports a mechanism involving an overall decrease in cortical epileptogenicity rather than an interruption of propagation pathways; callosotomy can produce a reduction in seizure severity and frequency without a transformation from generalized to partial seizures or, in some cases, complete suppression of seizure activity.14,58,61,62 Furthermore, in an intraoperative study of callosal compound action potentials and bilateral spike-and-wave discharges in epileptic patients, interhemispheric delay times were often less than the minimum axonal conduction time (approximately 20 milliseconds), and callosal compound action potentials peaked after their associated spike-and-wave complexes.63 In addition to the direct transfer of synchronous seizure activity, other mechanisms of corpus callosum–mediated modulation of epileptogenesis have been proposed. One hypothesis is that both hemispheres are capable of generating epileptiform discharges and that the corpus callosum provides a pathway for the synchronization rather than the transfer of activity.64 Consistent with facilitation via the corpus callosum, repetitive transcallosal volleys at 5 to 20 Hz enhance cortical reactivity as measured by thalamocortical responses in a rat model, an effect suppressed by callosotomy.65 It should be noted that callosal pathways are not required for seizure generalization; patients with Aicardi syndrome have congenital agenesis of the corpus callosum yet exhibit infantile spasms in addition to ocular abnormalities and mental retardation.66 In these patients, callosal pathways cannot mediate the propagation of synchronous activity from a lateralized focus, suggesting that seizure generalization must occur via other interhemispheric or subcortical tracts. From a wealth of animal studies, it appears that cortical and thalamic networks involved in physiologic sleep oscillations are critical in generating the spike-and-wave and poly-spike-and-wave complexes seen in certain generalized epilepsies, such as absence epilepsy and Lennox-Gastaut syndrome.67 A growing understanding of the behavior of interconnected networks indicates that the dynamic, oscillatory behavior of such systems is dependent on their topology, scale, and connectivity.68 The corpus callosum may comprise critical internodal connections of hemispheric thalamocortical circuits that provide the necessary dynamic system to generate generalized synchronous ictal activity. Corpus callosotomy has been demonstrated to be effective in the treatment of generalized seizures since as early as 1940.17 Multiple retrospective series show a reduction of generalized seizures after corpus callosotomy, with response rates ranging from 56 to 100%.61,69–72 The best and most consistent responses are seen in patients with drop attack seizures of tonic, atonic, or mixed type, with response rates approaching 100% in some studies.18,71,73,74 Similar response rates are seen in pediatric series,69,75,76 and callosotomy reduces medically refractory seizures in specific pediatric epilepsies such as the West and Lennox-Gastaut syndromes.77 In pediatric cases, an examination of parental satisfaction showed a high level of reported satisfaction with surgical outcome (88%), although interestingly satisfaction was not strongly correlated with measured seizure reduction and might have been related to behavioral changes, such as improved alertness and responsiveness.78 Reduction in seizure frequency is also correlated with perceived changes in quality of life, with improvements in self-care, family life, and school performance.79 Although the efficacy of corpus callosotomy for drop attacks is generally well accepted, the factors that contribute to a favorable outcome have been a topic of debate. There has been significant variation in the extent of callosal division used to achieve seizure control. Several studies have demonstrated that total corpus callosotomy is more efficacious and durable than partial divisions in the treatment of drop attacks.17,18,70,71,80–82 In general, better results are achieved with greater extents of resection, and often clinical algorithms can be developed for recommending a complete callosotomy in children who have severe underlying cognitive dysfunction or language impairment. Partial callosotomy limited to the anterior two-thirds of the callosum can provide some degree of palliation in children with good cognition and language as a first stage, but incremental benefit can still be gained with the conversion of partial procedures to total divisions if seizures continue.70,77 Recognition of an increased risk for disconnection syndrome after the disruption of posterior callosal fibers, either alone or as part of total callosotomy,72 led to the development of a two-stage procedure in which an initial anterior callosotomy is followed by a 6- to 12-month evaluation period to determine effectiveness before optional conversion to total resection.70 In terms of reduction of seizures, there are no observed differences between children and adults, and the outcome of either anterior or total callosotomy is not related to age at the time of surgery. Efforts to identify EEG predictors of responsiveness suggest that lateralized changes in interictal EEG can predict a good outcome of callosotomy,71 although specific patterns of ictal EEG activity may be a stronger prognostic factor.83 Other factors that may portend a favorable outcome include the absence of abnormality on magnetic resonance (MR) imaging and the absence of identified seizure etiology.84 Significant adverse effects are relatively rare with callosotomy, and standard neuropsychological and psychosocial assessments generally reveal little change compared with the premorbid condition.45 A precise characterization of neuropsychological effects is difficult; many patients have preexisting developmental delay, frequent seizures, and multiple antiepileptic drug regimens, and effective seizure control often can improve testing results. A characteristic acute postoperative neurologic syndrome is observed with mutism, nondominant arm and leg apraxia, bilateral Babinski signs, and urinary incontinence.45,46 These complications are almost always transient, with the mutism resolving typically within several weeks, and evidence suggests that intraoperative retraction and manipulation, rather than callosal division, may be the cause. Permanent side effects of callosotomy are relatively rare, with weakness or apraxia and impairment of language and behavior occurring in 8% of partial and 12% of total callosotomies.85 After callosotomy, dysphasia is seen specifically in patients with mixed or crossed cerebral dominance, in whom the language-dominant hemisphere does not control the dominant hand86; specifically, dysgraphia and dysphasia were seen in crossed-dominant right-handed patients, and dysgraphia alone was seen in crossed-dominant left-handed patients. Sensory disconnection resulting from the division of posterior callosal fibers is well documented, but the effects are subtle, and controlled neuropsychological testing is often required to identify it (see above). Disruption of the interhemispheric integration of motor information makes it more difficult for patients who have undergone a callosotomy to learn new bimanual tasks,48 but previously acquired bimanual tasks remain intact. Memory deficits are noted in a small subset of cases and may represent either preexisting extracallosal damage to structures like the fornix or extensive posterior callosal sections affecting the hippocampal commissures.87–89 Anterior callosotomy and single-stage complete corpus callosotomy are performed with the patient under general anesthesia. The patient is in the supine position, with the head elevated and very slightly flexed. Typically, we prefer a simple linear incision 2.5 cm in front of the coronal suture, extending from 3 cm to the left of midline to 7 cm to the right of midline, directly perpendicular to the midsagittal line (▶ Fig. 74.1). Two bur holes are placed right on the midline. One is at the bregma, the confluence of the coronal and sagittal sutures, or no more than 1 cm posterior to this point. The other is placed 5 cm anterior to the bregma, again directly at or just left of midline. To achieve adequate midline visualization along the corpus callosum, it is necessary that the bone flap come exactly to the midline or even slightly to the left of midline. The careful placement of bur holes directly over the sagittal sinus has proved to be a safe practice, but to avoid potential injury or any difficulty with midline visualization, the authors place the bur holes and the edge of the craniotomy slightly to the left of the sinus to avoid obstruction of the view by overhanging bone or injury to the sinus. A trapezoidal or D-shaped craniotomy that is 5 cm long is made with a high-speed drill. The dural opening needs be only 1.5 to 2 cm from the edge of the sagittal sinus and parallel to it. There are often bridging veins at the level of the coronal suture that require care in preservation as this dural opening is made. Venous lakes in the region also sometimes have to be oversewn. Although an experienced operator can visualize the entire corpus callosum through a smaller bone opening, we prefer approximately 5 cm of exposure here anterior to the coronal suture to provide flexibility in circumventing any bridging veins. Careful analysis of the vascular anatomy on MR imaging can be helpful in preparing for venous structures that may be encountered. After the dura is opened, the right frontal lobe is gently retracted away from the falx under the microscope. Fixed retraction is avoided on the brain itself but can be applied to the falcine side if necessary. There are occasionally significant arachnoidal adhesions, and below the lower limit of the falx there are almost always adhesions between the two cingulate gyri. Microscopic dissection is used to divide the arachnoidal adhesions. It is important not to mistake the callosal marginal arteries for the pericallosal arteries and therefore mistake cingulate gyrus for corpus callosum. The corpus callosum is significantly whiter and smoother than the cingulate gyrus, and generally one or both pericallosal arteries come into view at the same time as the corpus callosum. Fig. 74.1 (a) The child is positioned with the neck slightly flexed and the forehead toward the ceiling. A straight coronal incision 3 cm in front of the coronal suture permits a tight-shaped skull exposure. The craniotomy, in the shape of a large letter D, is begun with bur holes over the sagittal sinus just behind the coronal suture 6 cm in front of it. The left edge of this opening is just to the left of the sagittal sinus. (b) The placement of retractors through this precoronal bone flap allows exposure of the entire corpus callosum. Once the midline has been determined by identification of the raphe between the two ventricles, removal of the commissure or white matter can proceed rapidly anteriorly and posteriorly. The use of a microdissector passed just posterior to the anterior cerebral arteries allows the genu of the corpus callosum to be completely sectioned. This exposure also allows estimation of a point approximately two-thirds back in anterior corpus callosotomy (stippled area). (c) Detail of a ball-tipped micro nerve hook in the cavum vergae space that keeps the corpus callosum division on midline and prevents straying in either lateral ventricle. Next begins the phase of dissecting between the two pericallosal arteries to expose the corpus callosum over the entire length of the needed visualization. Usually, there is no doubt about which pericallosal artery is the right and which is the left, but if there is any variation from normal anatomy, it can be deciphered at this time by following small branches off these arteries either to the right or to the left. It is almost always worthwhile to dissect the two arteries apart so that the actual callosotomy can be made between them. If this is not possible without significant manipulation of the artery, it can also be arranged to do the callosotomy on one side or the other of both of the arteries, but this generally makes it more difficult to stay on the true midline as the artery crosses back and forth over the surgical field. Once the corpus callosum is well exposed from the genu to the body and the splenium, the desired posterior margin of the callosotomy can be determined through visual inspection and direct measurement and/or image guidance. The corpus callosum is entered in the midline at the level of the coronal suture (▶ Fig. 74.2). Even in a patient without a cavum septum evident on MR imaging, there is usually a small space between the two medial surfaces of the lateral ventricle and the corpus callosum, and the goal on coming through the corpus callosum is to enter through to this space, leaving the ependyma intact so as not to enter into the ventricle. Avoiding entry into the ventricles and preserving the ependyma significantly decreases complications related to intraventricular hemorrhage (including chemical meningitis and hydrocephalus). The midline is sometimes marked with a small vein, and when present this can be used as an anatomical guide. If there is inadvertent deviation from midline, the ependyma may come into view; it appears as a translucent layer with dark-appearing underlying ventricular fluid. If deviated from midline, effort should be made to explore slightly to the right and left to find this midline raphe. Once the raphe is located, a small, ball-tipped microdissector can be inserted into it, and this can be used to pull the corpus callosum up from below toward the aspirating sucker, dissecting forward toward the genu first and then posteriorly to the splenium later. It is generally possible to stay out of the ventricle entirely by using this maneuver. However, if the ependyma is breached, a small piece of collagen pad can be placed over the opening to prevent intraventricular passage of blood and debris. The callosotomy is continued anteriorly in the same plane into the genu, following the pathway and orientation of the pericallosal arteries. Once the anterior cerebral arteries are identified on the anterior aspect of the genu, the callosotomy can be safely continued all the way to the anterior communicating artery complex to the level of the anterior commissure, which is visible and deep and posterior to the genu at this point of the dissection. Fig. 74.2 Photographs through the operating microscope show exposure of the corpus callosum and identification of the midline raphe with corpus callosum division, as described. (a) Low-magnification and (b) higher-magnification views of the approach to the corpus callosum, which is distinctly whiter than the abutting cingulate cortex. (c) Aspiration of the callosal white matter shows the midline raphe between the ventricles, at the floor of the cavum vergae. Complete removal of the callosal fibers is ensured by proceeding forward with the ball-tipped micro nerve hook just above the raphe. (d) Further forward, the raphe falls away as the corpus callosum curves around the genu. The passage of a dissector between the superior part of the callosal fibers and the arachnoid allows the genu to be pulled posteriorly and divided, with direct visualization of the arachnoid down to the region of the anterior communicating artery complex. The decision about the posterior extent of disconnection is planned preoperatively based on the review of the sagittal MRI. The length of disconnection, measured from the anterior tip of the genu, is about two-thirds the length of the total corpus and avoids sectioning of the splenium. Image guidance techniques can be very useful in gauging the distance back on the corpus callosum in anterior callosotomy to avoid inserting modified disposable rulers or patties. The disconnection then proceeds posteriorly with the same technique used in the anterior division. After adjustment of the patient’s position head down with a small amount of Trendelenburg and of the angle of the microscope, the midline cleft between the ventricles is followed posteriorly to maintain a midline position and avoid injury to the corona radiata on either side of the corpus callosum. The cleft usually blurs posteriorly as the ventricles diverge from each other to the atria and then the temporal horns. The midline, though, can still be followed, and the posterior margin of the splenium (in the case of a total callosotomy) can be visualized. The callosotomy is completed through the splenium to the posterior commissure, with the arachnoid surrounding the vein of Galen left intact. After completion of the callosotomy, hemostasis is meticulously achieved. A piece of collagen pad is cut to the appropriate size and positioned in the callosotomy defect. The dura is then reapproximated, the bone flap replaced, and the wound closed with standard technique. Electrical stimulation for the treatment of seizures can be applied to various targets within the central and peripheral nervous systems. The goal of neurostimulation is to inhibit epileptogenicity; however, the mechanisms of action are poorly understood. Both open-loop and closed-loop systems are used for neurostimulation. Deep brain stimulation (DBS) and vagus nerve stimulation (VNS) are the two open-loop systems most commonly employed, in which continuous stimulation is applied according to set algorithms without regard to whether the patient is having a seizure or not. In contrast, closed-loop applications, such as regional neurostimulation (RNS), provide acute stimulation only when abnormal electrical activity is detected.90–92 The closed-loop algorithms required to detect the ictal state, as well as the electrical stimulation parameters necessary for seizure termination in both open- and closed-loop systems, are areas of active research.93–95 Closed-loop systems are dynamic circuits that use real-time feedback to deliver stimulation to a specific areas, either to the area of an epileptic focus or through circuit networks shared by the focus, only in a time of anticipated need. As a self-modulating system, RNS has the potential for tremendous sophistication. In its current state, RNS is of limited utility and can be applied only to definable epileptic foci. Fairly simple algorithms are used for RNS execution. Nonetheless, the techniques used for the detection of ictal activity and the delivery of electrical stimulation are areas of active research, and this is a technology that will certainly continue to evolve.93–95 Current evidence suggests that changes in the dynamics of electrical activity can be detected well before the development of seizures,90 thus allowing earlier stimulation and potentially more effective treatment. In contrast to a closed-loop system, an open-loop system like DBS applies continuous stimulation to deep brain nuclei without reference to the brain’s electrical activity. A wide variety of targets for the treatment of refractory seizures have been evaluated and include the cerebellum, basal ganglia, subthalamic nucleus, and thalamus (centromedian, anterior thalamic nuclei).96–101 Although the results of many of the studies show promise, definitive conclusions are often prohibited by small patient populations and a lack of long-term follow-up. Stimulation of the anterior thalamus, on the other hand, has been thoroughly studied. The randomized controlled SANTE (Stimulation of the Anterior Nucleus of the Thalamus for Epilepsy) trial demonstrated a median rate of seizure reduction of 56% by 2 years and a higher than 50% rate of seizure reduction in 54% of patients with medically refractory partial seizures.102 During the blinded phase of the study, patients with temporal lobe seizures showed a greater benefit than with those with extratemporal or multifocal seizure onsets. The prototypic neurostimulation procedure for epilepsy—and the only antiepileptic stimulation treatment currently approved by the Food and Drug Administration (FDA)—is VNS. VNS was first attempted in a human patient in 1988103 and was approved by the FDA in 1997 as an adjunctive treatment for medically refractory partial-onset epilepsy. The rationale for the intermittent unilateral stimulation of the cervical portion of the vagus nerve is based on the long-standing observation in laboratory animals that vagal stimulation produces EEG cortical desynchonization.104,105 A growing body of literature demonstrates that VNS is a safe, well-tolerated, and efficacious palliative treatment for both partial-onset and generalized medically refractory epilepsies. The vagus nerve (cranial nerve X) is a mixed motor and sensory nerve. Its wandering innervation extends from the medulla to the splenic flexure of the colon. Efferent fibers comprise only 20% the vagus nerve, carrying branchial motor efferents to the larynx and pharynx and parasympathetic visceral efferents to the pharynx, larynx, and organs of the thorax and abdomen.106 The remaining 80% of the vagus nerve carries primarily visceral afferent fibers from the neck, thorax, and abdomen and from stretch receptors in the aortic arch and chemoreceptors in the carotid bodies; a small proportion of fibers also carry somatic sensory (external auditory meatus, tympanic membrane) and special sensory (taste) afferents. Asymmetric development leads to an association of the right vagus nerve with the cardiac atria and of the left vagus nerve with the cardiac ventricles, with relatively less dense vagal innervation of the ventricles.107 This asymmetric cardiac innervation is the likely reason why unilateral stimulation of the left vagus nerve minimizes cardiovascular side effects in humans. Vagus nerve fibers distribute widely through the central nervous system, both monosynaptically and through the nucleus of the solitary tract (NTS).108 Although a small number of fibers connect directly to the spinal trigeminal nucleus and the reticular formation, the vast majority of vagal afferents are carried to the NTS via sensory neurons of the nodose ganglion. Three major output pathways distribute afferent vagal information from the NTS to dispersed central nervous system structures.109,110 The first pathway mediates feedback control of the heart rate (baroreceptor reflex) and respiration (Hering-Breuer reflex) via autonomic preganglionic and somatic motor neurons in the medulla and spinal cord, while the second pathway targets the reticular formation of the medulla and controls autonomic and respiratory reflexes.111,112 The third major NTS output pathway projects to forebrain structures via the parabrachial nucleus of the dorsal pons and is the most likely mediator of VNS anticonvulsant activity. The parabrachial nucleus has widespread projections to the insular, infralimbic, and lateral frontal cortex, as well as to the thalamus, hypothalamus, amygdala, and basal forebrain.113 Via the amygdala, output pathways from the NTS project to other limbic structures including the hippocampus and entorhinal cortex. Notably, there is direct parabrachial input to the intralaminar and midline nuclei of the thalamus, which project widely throughout the cerebral cortex and may influence both physiologic and pathologic cortical synchronization.114,115 Most cerebral structures are three or more synapses away from the vagus nerve; this relatively large distance between the brainstem vagal afferent system and the different cortical sites of epileptogenesis responsive to VNS suggests a role for systems projecting diffusely between the brainstem and forebrain. Various anatomical pathways are activated during VNS, but the specific circuitry responsible for anticonvulsant activity and the nature of the neurophysiologic changes (e.g., increased vs. decreased activity) remain unknown. Examination of fos gene product expression following brief intermittent VNS in rodents showed elevated neuronal activity in the amygdala and limbic neocortex (cingulate and retrosplenial cortex), both of which are implicated in epileptogenesis, as well as in hypothalamic and noradrenergic nuclei.116 Positron emission tomography (PET) neuroimaging studies have shown acute increases in regional cerebral blood flow to the medulla, right postcentral gyrus, and bilateral thalamus, hypothalamus, and insular cortex during VNS, with deactivation bilaterally in the hippocampus, amygdala, and posterior cingulate gyrus.117 A higher level of stimulation affected the same structures as the low-level stimulation, but with increased volumes of activation and deactivation, and additionally activated the bilateral orbitofrontal gyrus, right entorhinal cortex, and right temporal pole. In follow-up PET studies, only left and right thalamic activation was correlated with decreased seizure frequency after 3 months of VNS.118 More recently, functional MR imaging (fMRI) during VNS demonstrated robust activation of the thalamus and insular cortex, with post-VNS seizure reduction also associated with thalamic activation.119,120 The diffuse cortical effects of VNS can be observed on the EEG, as in the early studies, which showed cortical desynchronization in cats after cervical VNS.104,105 Animal studies further demonstrated that repetitive vagal stimulation can induce either synchronization or desynchronization of the EEG, depending on stimulation frequency and current strength.105,121,122 Specifically, high-frequency NTS stimulation (> 30 Hz) induces EEG desynchronization, whereas slower stimulation (< 17 Hz) induces synchronization.123 This preferential induction of synchronization with lower-intensity and slower-frequency stimulation and desynchronization with higher-intensity and faster-frequency stimulation may result from the differential recruitment of vagal afferent fibers, with desynchronization requiring activation of smaller-diameter unmyelinated fibers. In contrast, VNS in humans does not generally produce obvious or readily observable synchronization or desynchronization of the EEG.124,125 VNS produces evoked potentials of cerebral origin in scalp EEG only at stimulation intensities higher than those used clinically,126,127 but there is evidence that chronic VNS results in altered somatosensory and visual evoked potentials, which presumably reflect long-term changes in underlying neural circuitry.128,129 In addition to its effect on the underlying physiologic EEG, VNS has observable effects on pathologic interictal epileptiform discharges and seizure-like activity. The frequency of penicillin-induced focal interictal spikes in a rodent model of acute epilepsy is reduced 33% during VNS, an effect that can persist for minutes after the termination of electrical stimulation.130 VNS has similar abortive effects on acute chemically induced seizures in rodent and canine models, an effect dependent on the activation of unmyelinated vagal C fibers.130–132 Although VNS in humans produces no observable changes in the interictal scalp EEG,124 interictal epileptiform sharp waves recorded with hippocampal depth electrodes were decreased by 30-Hz vagal stimulation and increased by 5-Hz stimulation in a case report.133 VNS also has acute prophylactic anticonvulsant effects that extend beyond the duration of stimulation, decreasing the number and duration of pentylenetetrazol-induced seizures in rats for up to 10 minutes after the termination of stimulation.134 Furthermore, the prophylactic anticonvulsant effect of chronic VNS is progressive; chronic vagal stimulation over weeks is accompanied by decreasing seizure activity in an alumina gel primate model,135 and longer-term VNS (up to 12 months) in human patients induces initial clustering of EEG epileptiform activity followed by progressively increased spike-free intervals.136 Despite accumulating data, the precise mechanism underlying VNS-mediated anticonvulsant activity remains poorly understood. Antiepileptic drugs exert their effect through the modulation of voltage-dependent sodium and calcium currents and γ-aminobutyric acid (GABA)–mediated inhibition.137,138 Whereas antiepileptic drugs function by directly modulating cellular signaling on a molecular level, VNS functions through the stimulation of widely projecting brainstem pathways, which may result in the activation or inhibition of target regions. Thalamic nuclei are likely involved, given their consistent activation in PET and fMRI studies, the correlation between thalamic activation and seizure reduction, the diffuse nature of thalamocortical projections, and their role in physiologic and pathologic cortical synchronization.67,115,139 Noradrenergic activity also may be central to VNS function; noradrenergic nuclei show increase fos expression following VNS,116 and chemical lesion of the locus ceruleus significantly attenuates VNS-induced seizure suppression in rats.140 In VNS-implanted rats, it has been demonstrated that stimulation increases norepinephrine concentration in the prefrontal cortex.141 Other associated biochemical changes include increased expression of brain-derived neurotrophic factor (BDNF) and fibroblast growth factor (FGF) in the hippocampus and cerebral cortex, and decreased expression of nerve growth factor in the hippocampus. It has also been postulated that VNS affects seizure thresholds by modulating inhibitory neurotransmission; chronic VNS is associated with increased cerebrospinal fluid levels of the inhibitory neurotransmitter GABA, but these changes were not correlated to the degree of seizure reduction.142 VNS has been shown to be effective in managing both partial and generalized seizures, with the primary indicator of efficacy being a reduction of seizure frequency.143–145 In the multicenter randomized double-blinded controlled EO3 and EO5 studies, patients with medically refractory partial-onset seizures were implanted with a VNS system and received either a high-stimulation (30 Hz, 30 seconds on, 5 minutes off, 500-μs pulse width) or a low-stimulation (1 Hz, 30 seconds on, 90 to 180 minutes off, 130-μs pulse width) protocol for 12 weeks. Patients receiving the higher-stimulation treatment experienced mean reductions in seizure frequency of 24.5% and 28% in the two studies, compared with 6.1% and 15% in patients receiving the lower-stimulation protocol, but both the high- and the low-stimulation treatment produced statistically and clinically significant seizure decrement. In a nonrandomized treatment trial of 24 patients, VNS also reduced the frequency of generalized seizures by 46% after 3 months of stimulation.144 Although the current FDA indication for VNS covers patients 12 years and older, clinical data suggest that VNS has efficacy in younger pediatric patients as well. A study of 60 patients age 3 to 18 years (median age, 15 years; 16 patients younger than 12 years) demonstrated a 23% median decrease in seizure frequency after 3 months of stimulation, with a progressive benefit of continuing stimulation (31%, 34%, and 42% at 6, 12, and 18 months, respectively); patients had either partial or generalized tonic–clonic seizures (27%), and the response rate was not specific to seizure type.146 Likewise, an uncontrolled retrospective study of patients between 11 months and 16 years of age with complex partial or generalized (atonic, absence, tonic–clonic) seizures reported improvements in seizure reduction regardless of age and seizure type.147 VNS has also been used successfully to manage patients with Lennox-Gastaut syndrome. In 13 patients with Lennox-Gastaut syndrome (4 to 44 years of age), 6 months of VNS produced a 52% median reduction in seizure frequency, including 3 patients with a decrease of more than 90%.148 In a smaller study of pediatric patients with epileptic encephalopathies, there was a greater than 50% reduction of seizure frequency in 4 (27%) of 15 patients, and a significant improvement in perceived treatment side effects and general behavior was noted, although no significant group improvement in seizure frequency or severity, adaptive behavior, or EEG.149 In a recent randomized controlled study of 41 children with intractable epilepsy (35 with localization-related epilepsy and 6 with generalized epilepsy), VNS reduced seizure frequency by 50% or more in 26% of participants.150 In general, VNS has proved to be safe and well tolerated.151–153 The most commonly reported side effects include dysphonia (20%) and cough (6%), which most often occur during the stimulation phases and resolve during the quiescent phase of the stimulatory algorithm.8 In the randomized double-blinded EO3 and EO5 studies, only voice alteration was more frequent in the high-stimulation than in the low-stimulation population; less common adverse effects included throat pain, nonspecific pain, dyspnea, paresthesias, dyspepsia, vomiting, and infection, with adverse effects in these two studies rated mild or moderate 99% of the time and with no observed negative impact on cognition, affect, or coordination.143,145 The impact of VNS on mood and cognition was looked at more closely in a recent randomized clinical trial.154 Consistent with studies supporting a role of VNS for treatment-resistant depression, the authors found that VNS not only did not negatively impact mood or behavior but also led to a generalized improvement in mood for the entire group. The observed benefit was unrelated to the effect on seizure frequency. Furthermore, there is a low rate of surgical complications, with device failure (2.7%) and deep infection (3.5%) the most commonly observed in a retrospective study of pediatric patients undergoing device implantation.155 One of the authors (P.D.A.) ) has had less than a 2% incidence of infection and only a 0.4% incidence of deep infection requiring removal of the device. Erosion of the pulse generator through the chest wall can be a concern in children or debilitated patients with little subcutaneous fat but is a relatively rare occurrence that can be avoided with a subpectoral implantation. Furthermore, the next generations of devices are thinner and smaller, making this even less of an issue. Cardiovascular adverse effects are a theoretical concern given the cardiac efferents from the vagus nerve, but significant cardiac complications have not been observed with VNS. A small number of cases have been reported of transient bradycardia or ventricular asystole during intraoperative testing of the device leads (estimated occurrence rate of 0.1%); proper caution dictates the use of test stimulation before generator implantation and close cardiac monitoring during the procedure.156,157 Despite the variable effects on cardiac rhythm observed during stimulation,158 studies reveal no long-term cardiovascular effects involving arrhythmia or heart failure. During the preoperative visit, we have found it useful to address expectations and counsel families that the therapeutic response to VNS is difficult to predict. The median response is an approximately 50% reduction in seizures, but a wide spectrum has been reported, and responses can be better or worse. In terms of patient selection, it is critical that the neurosurgeon first ensure that the patient does not qualify for more definitive, potentially curable procedures, such as focal resection, because VNS is merely a palliative therapy. It is also important that certain diagnostic studies, such as high-field-strength MR imaging, which requires the body magnet to send or receive signal, and magnetoencephalography, may not be possible in many centers after the VNS device is inserted. If these tests are important as part of the continued work-up or ongoing evaluation, steps to schedule them before the actual implantation should perhaps be considered. Other parts of the diagnostic work-up, such as routine head coil MR imaging and EEG, and even invasive monitoring, can be done with the VNS device in place and functioning if necessary. Although several variations have been developed for placement of the VNS device, virtually the only absolutely necessary steps are to ensure that the electrode leads contact the correct nerve in the correct vertical orientation, and that the post-generator device be accessible to the patient for magnet activation and to the physician for reprogramming. In the initial descriptions of the procedures,116,117 a vertical incision over the carotid sheath was described, and a horizontal incision more than 4 cm below the clavicle for placement of the device was suggested. Improvements to increase comfort and cosmetic appearance after ultimate placement of the VNS device have been developed subsequently over the years. Examples include the use of a single longer incision both to access the nerve and to make a pocket for the device on the chest or lateral pectoral incision.118 We have found that the most satisfactory approach in children is begun by making both incisions in the direction of skin creases, which tends to minimize the amount of widening of the incision and gives a better overall cosmetic result (▶ Fig. 74.3). It also becomes possible to use incisions that are quite limited (as little as 2 cm in the neck and 2.5 cm in the axillary region), and to place the device under the pectoralis muscle rather than over the pectoralis muscle, particularly in very young or very thin patients. Most patients have adequate subcutaneous tissue to hide and cushion the device, especially with the newer small generators (Model 103 and beyond). Because the generation of a subcutaneous pocket is relatively trivial and the creation of a subpectoral pocket is more common in pediatric than in adult practice, this description focuses on subpectoral placement. Fig. 74.3 Steps in the placement of a vagus nerve stimulator. (a) With the patient supine, incisions are marked in the direction of Langer lines in the skin, arranged to allow access to the carotid sheath in the lower neck, and tunneled up from the axillary pocket to the neck incision. This positioning allows an approach to the neck from both sides of the operating table and an approach to the axilla from the patient’s left. (b) Short Cloward retractors provide visualization while the vagus nerve is dissected free from the carotid sheath. (c) The tunneling tool contains two concentric plastic tubes, the inner of which snugly fits the single-pin connector of the Model 303 or 304 leads. (d) The helices are placed around the nerve.
74.1 Disconnection Procedures for the Isolation of Epileptogenic Tissue
74.1.1 Multiple Subpial Transections
74.1.2 Functional Hemispherectomy
74.1.3 Corpus Callosotomy
Corpus Callosum: Anatomy and Function
Corpus Callosotomy: Mechanism of Seizure Prevention
Corpus Callosotomy: Outcomes
Corpus Callosotomy: Surgical Technique
74.2 Neurostimulation
74.2.1 Regional Neurostimulation
74.2.2 Deep Brain Stimulation
74.2.3 Vagus Nerve Stimulation
Vagus Nerve Anatomy and Physiology
Vagus Nerve Stimulation: Mechanism of Seizure Prevention
Vagus Nerve Stimulation: Outcomes
Vagus Nerve Stimulation: Presurgical Counseling
Vagus Nerve Stimulation: Surgical Technique
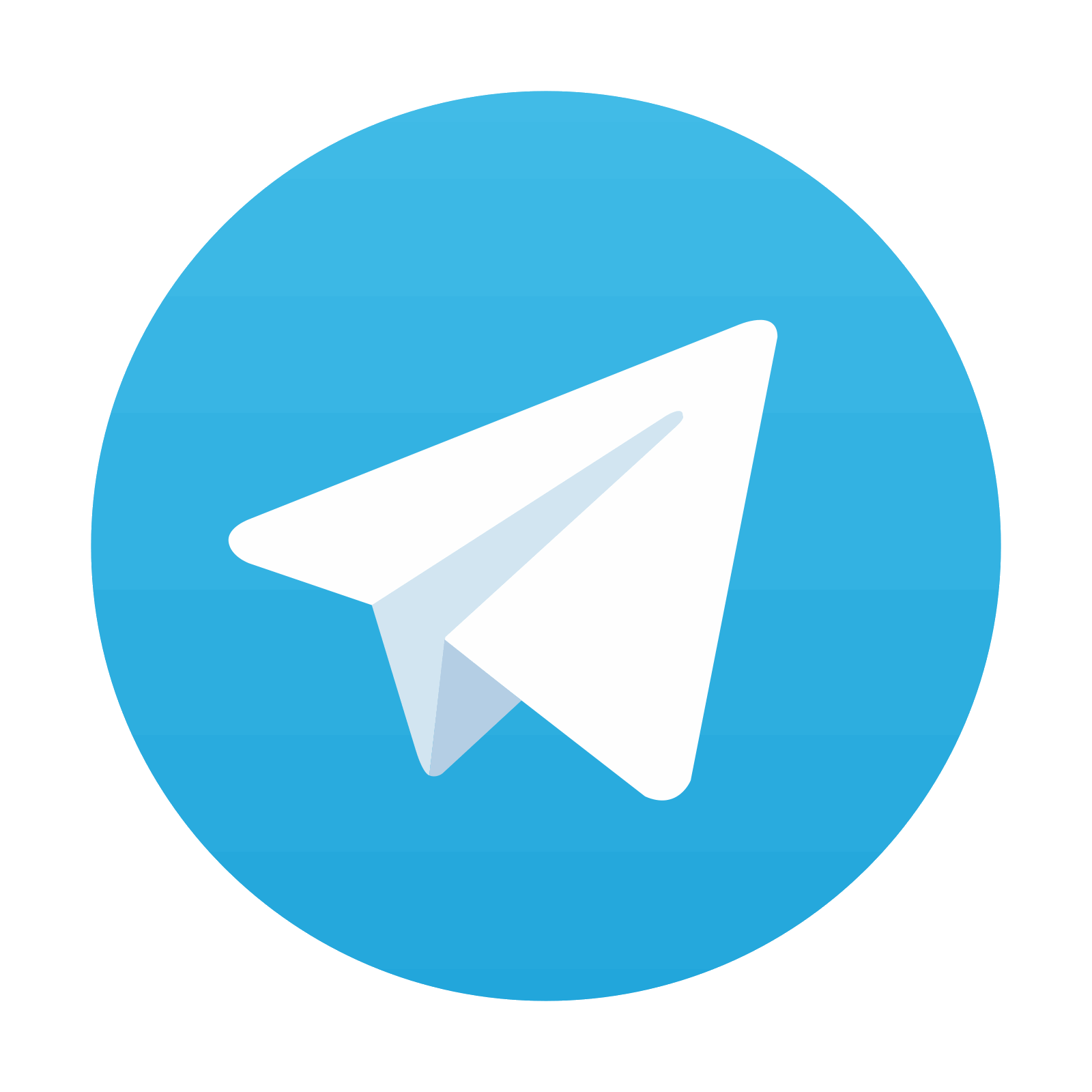