Carrier
Treatment
Phase
Study population
# of patients
Results
Reference
Nanoparticles
Aminosilane-coated iron oxide particles and alternating magnetic fields
I
Recurrent grade IV astrocytoma
14
• Minor or no side effects
• Median maximum intratumoral temperature of 44.6 °C
[15]
II
Recurrent grade IV astrocytoma
59
• Overall survival following first recurrence: 13.4 months
• Overall survival following primary diagnosis: 23.2 months
[16]
Liposomal doxorubicin
I
Pediatric patients with recurrent or refractory HGG
13
• No dose-limiting toxicities at 60 mg/m2
• Grade 4 neutropenia in 2 patients at 75 mg/m2
[17]
II
Recurrent HGG
13
• Disease stabilization in 54%
• Progression-free survival at 12 months: 15%
[18]
II
Newly diagnosed grade IV astrocytoma
40
• Progression-free survival at 6 months: 58%
• Median time to progression: 6.2 months
• Overall survival: 13.4 months
[19]
Liposomal cytarabine
II
Lymphomatous meningitis
30
• Only 1 (3%) recurrence
• 50% reduction in # of injections
[20]
II
Breast cancer with neoplastic meningitis
43
• Response (intent-to-treat): 21%a
• Median survival: 88 days
[21]
Rhenium nanoliposomes
I–II
Recurrent grade IV astrocytoma
N/Ab
N/Ab
NCT01906385
Viral vectors
Adenovirus-mediated p53
I
Recurrent HGG
12
• Maximum tolerated dose was not reached
• No evidence of systemic virus
• Transfected cells only within 5 mm of injection site
[22]
Recombinant adenovirus with the HSV TK gene followed by ganciclovir
I
Recurrent HGG
14
• Maximum tolerated dose was not reached
• Overall median survival: 4 months
• 4 patients survived >1 year
[23]
III
Newly diagnosed grade IV astrocytoma
236
• Median time to death or reintervention: 308 versus 268 days (p = 0.006)
• Overall survival: 497 versus 452 days (p = 0.31)
[24]
Stem cells
Neural stem cells with cytosine deaminase followed by oral 5-FC
I
Recurrent HGG
N/Ab
N/Ab
NCT01172964
Metallic nanoparticles are commonly composed of inorganic materials (such as gold, silver, or iron oxide) as well as metallic allotropes of non-metals (such as carbon fullerenes). Typically smaller than polymeric nanoparticles or liposomes, metallic nanoparticles are too dense to encapsulate a drug. Nevertheless, therapeutic agents can be successfully conjugated to the surface of metallic nanoparticles for delivery to the CNS. This approach was demonstrated successfully in a preclinical glioma model [11], and recent work has shown that the application of an external magnet can further enhance the ability of the particles to cross the BBB [25]. At the clinical level, therapeutic applications of metallic nanoparticles have been primarily limited to intracranial thermotherapy [15]. In a phase II study [16], aminosilane-coated iron oxide nanoparticles were injected into the tumors of 59 patients at 2 medical centers and exposed to an alternating magnetic field, resulting in heating of the particles. Although the patients tolerated the treatment well, other technologies including magnetic resonance imaging (MRI)-guided laser interstitial thermal therapy (LITT) and MRI-guided focused ultrasound (MRgFUS) offer competing options for achieving thermal ablation.
Polymeric nanoparticles, on the other hand, are capable of carrying a wide variety of payloads, including chemotherapeutic drugs, proteins, nucleic acids, and contrast agents. Polymeric nanoparticles are characterized by a large surface area containing functional groups that can be conjugated to peptides, antibodies, or other biomolecules. The ability to tailor these surface characteristics, as well as the physicochemical properties of the nanoparticle core, makes polymeric nanoparticles popular vehicles for drug delivery to the brain [26, 27]. In particular, stabilizers such as polysorbate 80 and polyethylene glycol (PEG) can be used to coat the surface of drug-loaded nanoparticles, thereby shielding the particles from clearance and extending their circulation time [28]. Various chemotherapeutic agents, including doxorubicin [29], camptothecin [30], and paclitaxel [31], have been encapsulated within polymeric nanoparticles for the treatment of brain tumors in preclinical studies, and phase I and II studies [32, 33] have begun to explore their safety and efficacy in patients with other types of solid tumors. Nevertheless, clinical trials involving patients with brain tumors are currently lacking.
Liposomes are nanoscale, self-assembled vesicles comprised of amphiphilic phospholipids that mimic the lipid bilayer of the cell membrane. As a result, liposomes are capable of carrying hydrophilic, hydrophobic, and amphoteric drug molecules and are effective at crossing the BBB [34]. Similar to polymeric nanoparticles, the surface properties of liposomes can be modified to extend their circulation time. Conjugation to hydrophilic polymers sterically stabilizes the liposomes, allowing them to avoid opsonization and uptake by the reticuloendothelial system [35]. Liposomal doxorubicin, in particular, has been used to treat patients with recurrent and/or refractory high-grade glioma (HGG) [17–19], as well as patients with brain metastases from solid tumors [36]. Other applications of liposomal delivery that are being actively explored include the intrathecal delivery of liposomal cytarabine for the treatment of neoplastic and lymphomatous meningitis [20, 21], as well as the intratumoral delivery of rhenium nanoliposomes—a novel form of brachytherapy—for the treatment of recurrent HGG (NCT01906385).
Through surface modifications and ligand conjugation, nanoparticles and liposomes that specifically target brain tumors have been developed. These nanotherapeutic agents are targeted to cell surface receptors that are expressed specifically by tumor cells, such as the epidermal growth factor receptor (EGFR) [37] and interleukin-13 (IL-13) receptor [38]. Recently, fibroblast growth factor-inducible 14 (Fn14), a member of the tumor necrosis factor receptor (TNFR) superfamily, has emerged as a novel target for GBM therapy [22]. While Fn14 levels remain low in normal brain tissue, the receptor is highly expressed by HGG—particularly the invasive cells at the rim of the tumor [39]. Nanoparticles coated with both PEG and ITEM4—a monoclonal antibody that recognizes the Fn14 receptor—were shown to specifically target tumor cells in a human xenograft model, with minimal non-specific binding to non-tumoral tissue [23]. Targeted therapeutics have the potential to reduce treatment toxicities and generate fewer side effects.
Viral Vectors
Viral vectors are biologic vehicles that have been studied extensively in the context of gene therapy, due to their innate ability to enter cells and transfer genetic material. While CNS-directed gene therapy is commonly considered a potential treatment for congenital disorders [24] and neurodegenerative diseases [40], applications in neuro-oncology have been explored as well. In particular, phase I studies have evaluated the safety and feasibility of using adenovirus as a viral vector for the delivery of p53 [41] and interferon beta [42] to patients with HGG. A particularly promising approach involves the intratumoral administration of a recombinant adenovirus carrying the herpes simplex virus (HSV) thymidine kinase (TK) gene followed by the intravenous injection of ganciclovir in patients with recurrent HGG [43]. The TK gene sensitizes the tumor cells to ganciclovir, thereby limiting the toxic effect to the neoplastic cells infected by the virus. This approach was tested in a recent phase III study [44] involving 236 patients at 38 centers in Europe, which identified a longer median time to death or reintervention in the experimental group (308 days vs. 268 days, p = 0.006), although overall survival remained unchanged.
Despite the advantages of viral vectors for the application of gene therapy, safety and toxicity concerns persist. Off-target biodistribution and insertional mutagenesis carry the risk of transgene overexpression, tissue damage, and tumorigenesis. Immunogenicity represents another obstacle, as clearance of the vector by the immune system reduces its efficiency as a delivery vehicle. Of note, adenovirus-associated virus (AAV) is being promoted as a safer option for viral delivery as it is non-pathogenic and non-autonomous—it can only replicate in the setting of coinfection with helper viruses. While this results in greater safety and fewer side effects, a limited amount of genetic material can be packaged within AAV. Further modification of viral vectors, as well as non-viral alternatives, is being explored for therapeutic delivery to brain tumors.
Immune and Stem Cells
As early as the 1980s, researchers recognized that immune cells are capable of crossing an intact BBB and subsequently infiltrating brain metastases [45, 46]. As a result, macrophages have been studied as a potential carrier for the delivery of nanoparticles to an experimental brain metastasis model [47]. Recently, however, focus has shifted toward the use of stem cells as alternative cellular vehicles. Neural stem cells [48] and mesenchymal stem cells [49, 50] cross the BBB effectively and migrate to primary and metastatic brain tumors, likely in response to cytokines and chemokines secreted by the tumor microenvironment. Neural stem cells, in particular, demonstrate a strong tropism for the tumor border, invasive glioma cells, and glioma stem cells [48]. Additionally, neural and mesenchymal stem cells locally suppress the immune system, thus evading immune recognition and rejection [51, 52].
Numerous preclinical studies have harnessed these unique, intrinsic features of neural and mesenchymal stem cells to deliver various therapeutic “cargo” to malignant brain tumors. Oncolytic viruses [53–55], drug-loaded nanoparticles [56, 57], and cytokines including tumor necrosis factor apoptosis-inducing ligand (TRAIL) [58–61], bone morphogenetic protein 4 (BMP4) [62], and interleukin-12 (IL-12) [63] have all been packaged within stem cells in order to enhance their delivery to tumor cells. One promising approach utilizes stem cells to deliver an enzyme—cytosine deaminase (CD)—to glioma cells, where it converts the inactive prodrug 5-fluorocytosine (5-FC) into the active chemotherapeutic compound 5-fluorouracil (5-FU) [64]. While 5-FC crosses the BBB efficiently following systemic administration, the natural tropism of stem cells for glioma cells ensures that the active drug will only be produced at sites of active tumor infiltration, thereby limiting its effect on healthy tissue. Preclinical studies [65] demonstrating the safety and efficacy of this stem cell-mediated enzyme/prodrug approach resulted in the first human study (NCT01172964) to use stem cells as a delivery vehicle for the treatment of HGG. Patients with recurrent HGG underwent intracranial injections of neural stem cells transduced with CD at the time of tumor resection or biopsy. Beginning four days later, the patients were administered oral 5-FC every 6 h for 7 days. Various doses of neural stem cells and 5-FC were tested for safety and feasibility, opening the door to further exploration of stem cell-mediated delivery for the treatment of malignant brain tumors.
Several limitations must be overcome before cell-mediated drug delivery can achieve broader use in patients. One technical challenge involves maintaining the viability of the cellular vehicle itself when dealing with cytotoxic cargo. Toxic effects of the therapeutic agent on the cell may result in premature cell death and drug release before the cell reaches its target. Nanoparticle formulations have therefore been used as a means of shielding the cell from its cargo and delaying cell death [66, 67]. Nevertheless, the lysosomal degradation of nanoparticles following endocytosis remains an issue. Spatial and temporal control over drug release from a cell vehicle therefore requires additional research. Further challenges arise from the poor loading efficiency of most cellular carriers, necessitating the delivery of large quantities of cells in order to achieve a therapeutic effect.
The largest obstacle to the clinical translation of cell-mediated delivery remains safety. Stem cells are defined, in part, by their capacity for self-renewal, which has raised concerns that stem cell therapy may induce malignant transformation. The spontaneous transformation of bone marrow-derived mesenchymal stem cells in long-term cultures has been a controversial issue [68, 69]. However, a clinical trial [70] involving the intracerebellar and intrathecal administration of neural stem cells for the treatment of ataxia telangiectasia identified one subject who developed a multifocal glioneural tumor 4 years after the treatment. Additionally, cytokines secreted by stem cells, in addition to their locally immunosuppressive effect, have been shown to promote tumor growth and metastasis in other tumor types [71, 72]. These safety concerns must be fully investigated for the success of cell-mediated delivery as a treatment strategy.
Routes of Delivery
Each of the drug carriers described above may be delivered to malignant brain tumors via several routes. Systemic administration must be coupled with strategies to cross the BBB, while other routes allow the BBB to be bypassed entirely.
Systemic Delivery
Current Strategies for Delivery Across the BBB
Numerous approaches for enhancing systemic drug delivery to the CNS have been developed over the past several decades, including intra-arterial therapy, osmotic BBB disruption, chemical BBB disruption, and manipulation of the endogenous RMT pathway. Intra-arterial delivery results in higher local drug concentrations than intravenous administration. The drug is distributed throughout the tumor capillary network and achieves a higher “area under the curve” due to the avoidance of first-pass metabolism by the liver [73]. Although typically, intra-arterial delivery is accomplished via the carotid artery, more recently cerebral angiographic techniques have been used to perform superselective intra-arterial infusions of chemotherapeutic agents [74]. Nevertheless, the administered agent must still cross the BBB in order to achieve its effect.
Transport across the BBB may occur via paracellular or transcellular routes. Osmotic BBB disruption, first proposed by Stanley Rapoport in the 1970s [75], is one mechanism for enhancing paracellular transport across the BBB. An intra-carotid infusion of a hyperosmotic agent (most commonly mannitol) is coadministered with an intra-arterial chemotherapeutic agent. The osmotic agent draws water out of the endothelial cell, produces vasodilation, and stimulates endothelial cell cytoskeletal contraction via a cadherin-dependent mechanism [76]. These processes place mechanical stress on the tight junctions linking the endothelial cells. While osmotic BBB disruption has demonstrated promising results in clinical trials [77], the technique has been associated with transient cerebral edema [78]. Additionally, non-specific disruption of the BBB takes place in areas of normal brain tissue, allowing plasma proteins to pass from the bloodstream into the parenchyma with resulting neurotoxicity [79].
Chemical BBB disruption offers a second mechanism for enhancing paracellular transport across the BBB. Intra-arterial vasoactive agents produce inflammation and destabilization of the endothelial cell membrane, resulting in transient opening of the BBB. Bradykinin, which binds to B2 receptors on the endothelial cell membrane and activates nitric oxide synthase, is a commonly studied agent for BBB disruption, with evidence suggesting that it specifically affects the BBB in the region of a brain tumor [80, 81]. More recently, RMP-7—a synthetic bradykinin analog—has been used due to its higher potency, longer half-life, greater specificity for the B2 receptor, and increased stability, enabling it to be delivered intravenously [82]. However, while phase I and early phase II studies [83, 84] found the combination of RMP-7 and carboplatin to be safe and effective for the treatment of refractory brain tumors, the treatment was ultimately ineffective in a larger, multicenter, placebo-controlled trial [85].
An alternative strategy for crossing the BBB involves molecular Trojan horses, which utilize the endogenous RMT pathway. Recombinant proteins or “chimeric” peptides are formed by linking a therapeutic agent that cannot typically cross the BBB on its own with a ligand or monoclonal antibody that recognizes a receptor on the endothelial cell surface. Upon binding to the receptor, the entire complex undergoes transcytosis [86]. Antibodies that recognize the transferrin receptor [87, 88] and the insulin receptor [89], both of which are found on cerebral endothelial cells, have been fused to diagnostic and therapeutic agents for delivery across the BBB in preclinical studies. Additionally, a phase I dose escalation study [90] was recently completed in which GRN1005/ANG1005, comprised of paclitaxel conjugated to a low-density lipoprotein receptor-related peptide, was administered to patients with recurrent HGG. While patients experienced toxicities including neutropenia and mucositis, there were no toxicities specific to the CNS. Furthermore, therapeutic concentrations of the drug were found in resected tumor tissue, suggesting effective transport across the BBB. Phase II trials (NCT02048059 and NCT01480583) involving patients with metastatic breast cancer are ongoing.
Ultrasound-Mediated BBB Disruption
MRI-Guided Focused Ultrasound: The Clinical Experience
Focused ultrasound is rapidly emerging as a promising tool for noninvasive disruption of the BBB. Interestingly, ultrasound was used for its therapeutic potential long before it was developed into the imaging modality that we recognize today. As early as the 1950s, Ballantine and colleagues [91] used an ultrasound beam to open the BBB in brain tissue without causing a lesion. However, the variable thickness of the skull creates beam distortions and attenuation, and prior to the 1990s, focused ultrasound could only be applied through an opening in the skull. More recently, hemispheric phased arrays of ultrasound transducers were designed together with computer software that predicts and corrects for the phase aberrations caused by the skull, enabling noninvasive focusing of the beam at points deep within the brain [92]. In addition, advanced imaging modalities—in particular, magnetic resonance thermometry—provide real-time monitoring of temperature changes at the skull, along the beam path, and at the focal point, allowing appropriate adjustments to be made [93].
Focused ultrasound produces a high rate of energy deposition at the focal point, resulting in spatial intensities that are orders of magnitude higher than in the prefocal region. Heat is released, and temperatures can reach up to 60 °C, at which point coagulative necrosis occurs [94]. This mechanism is being harnessed in clinical trials for the noninvasive ablation of deep brain targets in the thalamus and basal ganglia for the treatment of essential tremor and Parkinson’s disease. However, ultrasound also produces non-thermal, mechanical effects, including cavitation, acoustic radiation forces, and acoustic streaming. Cavitation refers to the oscillation of micron-sized gas-filled bubbles in response to the positive and negative components of the ultrasound beam. The diameter of the bubbles varies with the pressure field—at lower amplitudes, stable oscillation (i.e., non-inertial cavitation) occurs, but as the pressure amplitude increases, the bubbles become unstable and collapse (i.e., inertial cavitation). The latter process produces shock waves and high-velocity jets, which place mechanical stress on the adjacent tissue [95]. Acoustic radiation forces refer to the unidirectional forces that occur along the beam path as a result of the momentum that is transferred from the ultrasound beam to a reflecting or absorbing surface [96]. Radiation forces that occur in a liquid medium produce acoustic streaming, which may enhance convection [97].
Whereas continuous ultrasound exposures are typically used for thermal ablation, pulsed exposures with short duty cycles apply ultrasound energy at lower rates, producing temperature elevations of only 4–5 °C, allowing the mechanical effects of ultrasound to play a larger role. Kullervo Hynynen, Nathan McDannold, and colleagues [98] showed for the first time in 2001 that focused ultrasound could be used to noninvasively disrupt the BBB without damaging the surrounding tissue. Importantly, they introduced the concept of using intravenous lipid or albumin-encased gas microbubbles, 1–5 μm in diameter. The microbubbles cluster near capillary walls, where they undergo stable cavitation in the presence of low frequencies and pressure amplitudes. The resulting oscillations place stress on the endothelial tight junctions that form the BBB, localizing the effects of ultrasound to the vasculature at the focal point [99]. Microbubbles lower the threshold for cavitation and reduce the amount of energy needed to achieve BBB opening, thereby minimizing the risk of heating at the skull or damage along the beam path. In addition to the paracellular route generated by microbubble cavitation, recent evidence suggests that sonication may also promote transcellular transport across the BBB by upregulating proteins involved in transcytosis, such as caveolin-1 and caveolin-2 [100]. The resulting disruption of the BBB typically persists for 4–6 h [101].
Numerous preclinical studies have demonstrated that focused ultrasound can be used to facilitate the delivery of various agents across the BBB, including liposome-encapsulated doxorubicin [102–104], methotrexate [105], carmustine [106], temozolomide [107], and the monoclonal antibody trastuzumab [108, 109]. Immunotherapy agents (e.g., interleukin-12) [110], stem cells [111], and gene therapy vectors [112–114] have been successfully delivered across the BBB with ultrasound technology, as well. Ultrasound-induced opening of the BBB was demonstrated to be safe in two recent studies [115, 116] involving repeated BBB disruption in the central visual field targets and basal ganglia of non-human primates. Long-term neurological deficits were not identified.
The first phase I study (NCT02343991) of MRgFUS for opening of the BBB is currently ongoing. Brain tumor patients are administered microbubbles and undergo sonication, followed by the intravenous administration of liposomal doxorubicin. The patients are subsequently taken to the OR for resection of the tumor, with careful tissue sampling in the region of ultrasound application to measure the concentration of doxorubicin as well as in adjacent regions. The first patient to enroll in the study was recently treated successfully, without adverse effects.
Limitations of Focused Ultrasound
The potential for focused ultrasound to open the BBB noninvasively is particularly attractive. Nevertheless, transcranial focused ultrasound currently requires the application of a stereotactic frame, similar to deep brain stimulation and Gamma Knife radiosurgery. Although this can be performed on an outpatient basis, the inherent risks of any frame-based procedure apply, including pin site infection. In contrast to focused ultrasound ablation for the treatment of movement disorders, which entails only a single treatment, current chemotherapy regimens typically involve complex dosing schemes. Given the transient nature of ultrasound-induced BBB disruption, sonication would be required prior to each individual treatment—a complicated matter when a stereotactic frame is involved. Furthermore, the need for complete hair removal in order to achieve acoustic coupling is a source of hesitation for some patients, and the use of MRI to localize and monitor the targeted region precludes patients with pacemakers and certain metallic implants. Technological limitations exist, as well—while transcranial focused ultrasound has been optimized to treat small, focal targets in deep brain regions such as the thalamus and basal ganglia, it remains to be seen whether regions adjacent to the skull can be included within the treatment envelope, as well as whether the BBB can be opened in large volumes of brain tissue, such as the 2-cm rim surrounding a brain tumor, within a reasonable time frame.
Future Directions
In addition to addressing the limitations described above, researchers have been developing novel microbubbles to be used with focused ultrasound. The surface of a microbubble can be modified with ligands that recognize and bind to tumor-specific antigens, thereby increasing the specificity of the microbubble for tumor vessels. Additionally, a microbubble can be used to encapsulate a therapeutic agent, only releasing the drug upon exposure to ultrasound. As a proof of principle, carmustine-loaded microbubbles were recently shown to facilitate improved circulation and reduced clearance of the drug and improved survival in a murine tumor model [117, 118]. A vascular endothelial growth factor (VEGF) ligand was subsequently conjugated to the drug-loaded microbubbles, resulting in targeting of the drug’s effects to regions of tumor-induced angiogenesis [119]. Further advances in drug carriers, combined with ultrasound-induced BBB opening, will contribute significantly to effective drug delivery to malignant brain tumors.
Intrathecal and Intraventricular Delivery
As an alternative to opening or modulating the BBB, bypassing the BBB itself has become a common strategy. Early attempts to circumvent the BBB as well as the blood–cerebrospinal fluid (CSF) barrier involved delivering therapeutic agents directly into the CSF via the lumbar arachnoid space (intrathecal delivery) or ventricular system (intraventricular delivery). Intra-CSF administration is typically accomplished via lumbar puncture or through an indwelling, subcutaneous ventricular access device, such as an Ommaya or Rickham reservoir. However, rapid CSF turnover, as well as limited diffusion across the ependymal layer and into interstitial spaces, limits the efficacy of intrathecal or intraventricular administration for the treatment of parenchymal disease [120]. As a result, drug delivery to the CSF is generally reserved for treating meningeal diseases, including neoplastic meningitis and meningeal gliomatosis [121–125].
Intranasal Delivery
Intranasal Delivery: The Clinical Experience
Another route that has been garnering attention for its potential to noninvasively bypass the BBB involves intranasal delivery. Intranasal administration has been investigated previously as a means of achieving systemic distribution, due to the porous endothelial membrane and large surface area of nasal blood vessels, the rapid onset following administration, and the ability to avoid first-pass metabolism [126]. More recently, however, nasal administration has also been recognized as a strategy for delivering therapeutic agents directly to the CNS.
Following intranasal administration of a substance, transport must first take place across the olfactory and respiratory epithelia in the nasal passages. This may occur via intracellular pathways (including endocytosis into the olfactory sensory neurons and transcytosis across sustentacular cells) as well as extracellular pathways involving paracellular transport to the lamina propria. Paracellular transport may be facilitated by cell turnover within the nasal epithelium, which produces rearrangements of the epithelial tight junctions [127]. Local mechanical injury occurring during intranasal delivery may also allow larger substances to cross the nasal mucosa [128]. Substances that reach the lamina propria are then absorbed into the systemic circulation, drain via nasal lymphatics to the deep cervical lymph nodes, or travel along olfactory and trigeminal nerve endings to the olfactory bulb or the brain stem, respectively. Recent work examining the transport rate of [125I]-insulin growth factor-1 (IGF-1) from the nares to the CNS has suggested that substances are carried by bulk flow within the perineural spaces of the olfactory and trigeminal nerves to points of entry into the brain [129]. From there, the substances distribute to distant sites within the CNS via communication with the CSF of the subarachnoid space or bulk flow within the perivascular spaces of cerebral blood vessels, a process driven by arterial pulsations related to the cardiac cycle [130, 131].
A number of preclinical studies have explored the intranasal administration of various therapeutic agents for the treatment of malignant brain tumors. Carboplatin-loaded polycaprolactone nanoparticles [132], methotrexate [133], neural stem cells [134], mesenchymal stem cells [135], telomerase inhibitors [136], and small interfering RNA-based therapies [137] have been successfully delivered intranasally in various murine models of glioma. In humans, intranasal delivery has primarily been studied for cardiovascular, endocrine, and neurocognitive agents, including vasopressin [138], angiotensin II [139], cholecystokinin [140], insulin [141], and oxytocin [142]. The only known study involving an antitumoral therapy was a phase I–II study [143] that examined the intranasal delivery of perillyl alcohol, a compound with previously identified anticancer activity through a poorly characterized, transforming growth factor-β (TGF-β) and/or Ras-dependent mechanism. Perillyl alcohol had been shown to have some efficacy in other solid tumors, but oral administration resulted in a variety of gastrointestinal side effects [144, 145]. Adults with recurrent HGG were administered an inhalational formulation of perillyl alcohol 4× daily through the nose. None of the patients in the study reported any toxicities associated with the treatment. However, further studies will be needed to study the efficacy of this treatment.
Limitations of Intranasal Delivery
One challenge in the study of intranasal delivery is that small animal models are not particularly representative of nasal anatomy and physiology in humans. Approximately 50% of the total surface area of the nose consists of olfactory epithelium in rats, whereas it is only 3–8% of the total surface area in humans [128]. Therapeutic agents that were successfully delivered to the CNS via the intranasal route in murine models may therefore fail in clinical trials. Other limitations are related to the low pH and relatively low permeability of the nasal mucosa. As a result, intranasal drug delivery is mainly limited to potent drugs that are delivered in small volumes. Higher volumes are likely to cause nasal irritation or injury. Active mucociliary clearance represents yet another obstacle to efficient intranasal delivery [127]. New drug formulations and carriers are therefore being explored in order to optimize the use of the intranasal route for the treatment of malignant brain tumors.
Interstitial Delivery
While intrathecal, intraventricular, and intranasal delivery bypasses the BBB, interstitial delivery represents the most direct option for intraparenchymal treatment. The current options for the interstitial delivery of therapeutic agents include implantable interstitial wafers and catheter-based convection-enhanced delivery (CED).
Interstitial Wafers
Carmustine Interstitial Wafer: The Clinical Experience
In 1976, Robert Langer and Judah Folkman revolutionized the drug delivery world by successfully incorporating macromolecules into non-inflammatory, biodegradable polymers [146]. These polymers can be safely implanted into biologic tissues, where subsequent degradation facilitates the sustained release of the biochemically active macromolecules. By modifying the chemical composition of the polymer, the kinetics of polymer degradation and macromolecule release can be closely regulated. Throughout the 1980s and 1990s, Langer partnered with Henry Brem and his colleagues at the Johns Hopkins University in order to translate this work from the bench to the operating room. Their efforts culminated in the clinical application of interstitial chemotherapy in the form of carmustine-loaded wafers, which currently remains the only FDA-approved locally delivered treatment for HGG.
The carmustine interstitial wafer (CIW) is comprised of the polyanhydride polymer poly [1,3-bis-(p-carboxyphenoxy propane)-co-(sebacic anhydride)] (CPP:SA) loaded with 3.8% carmustine (bis-chloroethylnitrosourea (BCNU)), a member of the nitrosourea family of chemotherapeutic agents [147]. At the time that CIW was being developed, intravenous BCNU was a standard treatment for HGG at many centers. The systemic administration of nitrosoureas had been shown to have a modest effect on survival [148], but BCNU has a short half-life and has been associated with side effects that include pulmonary fibrosis, myelosuppression, and hepatic dysfunction [149]. BCNU therefore represented a good choice to be incorporated into the hydrophobic matrix of the wafer, which would increase its half-life, increase local delivery of the drug to the residual tumor cells at the margin of the resection cavity, facilitate sustained release of the drug over 2–3 weeks, and decrease off-target effects.
Initial clinical trials [147] examined CIW implantation in patients with recurrent gliomas who had completed radiation therapy. A phase I–II study enrolled 21 patients with recurrent grade III or grade IV astrocytomas, who underwent tumor resection followed by implantation of up to 8 wafers. Three concentrations of BCNU were studied: 1.93, 3.85, and 6.35% by weight. Patients tolerated the interstitial treatment at all 3 doses, and there were no adverse reactions to the BCNU wafers themselves. The intermediate dose of BCNU, 3.85% by weight, was studied in a subsequent phase III study [150]—a prospective, randomized, placebo-controlled trial involving 27 centers and a total of 222 patients with recurrent grade III or grade IV astrocytomas. The study demonstrated a median survival of 31 weeks versus 23 weeks for patients receiving CIW versus placebo, respectively (hazard ratio = 0.67, p = 0.006), and the 6-month survival of patients with GBM was 50% higher in the treatment group relative to placebo (p = 0.02). The following year, in 1996, the FDA approved CIW for patients with recurrent HGG.
A second set of clinical trials focused on the use of CIW at the time of initial diagnosis. A phase I study [151] involving 21 patients with grade IV and 1 patient with grade III astrocytoma established the safety of implanting CIW during the initial tumor resection, followed by radiation therapy. A phase III prospective, multicenter, randomized, double-blind trial [152] involving 32 patients identified a median survival of 58 weeks versus 40 weeks for the treatment and placebo groups, respectively (p = 0.012). A subsequent phase III study [153] confirmed the survival benefit in a larger group of patients, enrolling 240 patients and establishing a median survival of 13.9 months in the treatment group versus 11.6 months in the placebo group, with a 29% risk reduction (p = 0.03) over the course of 30 months. A follow-up study [154] confirmed that on long-term follow-up (i.e., 3 years after implantation), the survival advantage was maintained. The success of these clinical trials prompted the FDA to approve CIW for patients with newly diagnosed HGG in 2003.
CIW was the first major advance in HGG therapy since 1978, when radiation therapy was adopted into the standard adjuvant treatment of HGG [149]. In 2005, however, a second agent was added to the neuro-oncologist’s armamentarium, when a phase III study by Roger Stupp and colleagues [155] demonstrated the safety and efficacy of a postoperative chemoradiation treatment strategy using orally delivered temozolomide and fractionated radiation therapy together over 6 weeks followed by maintenance temozolomide for six months. This regimen improved median survival from 12.1 to 14.6 months compared to radiation alone. Following this study, clinicians and researchers have explored the safety and efficacy of combining CIW with postoperative radiotherapy and temozolomide. Typically, chemoradiation is not initiated until 2–4 weeks following surgical resection or biopsy to allow for recovery and healing; CIW therefore offers a theoretical bridge to the chemoradiation treatments, providing sustained release of BCNU during the intervening period. A number of retrospective studies [156–163] and two prospective, observational studies [164, 165] have supported a role for multimodal therapy and have encouraged efforts for a randomized phase III study.
Limitations of Interstitial Wafers
Despite the survival advantage offered by CIW, some clinicians have been hesitant to implement interstitial wafers into their practice due to concerns that there is an increased risk of perioperative complications. Theoretical risks of CIW include seizures, cerebral edema, intracranial abscess, CSF leak, wound infection, impaired wound healing, and wafer migration. The phase III study that established the role of CIW in the initial treatment of HGG found similar rates of adverse events in the treatment and placebo arms of the trial, with two exceptions: CSF leak (5% in the treatment group vs. 0.8% in the placebo group) and delayed intracranial hypertension (9.1% in the treatment group vs. 1.7% in the placebo group) [153]. As a result, CIW has only been recommended for circumstances in which a watertight dural closure can be obtained; additionally, patients are monitored for cerebral edema and treated with high-dose corticosteroids postoperatively. Nevertheless, reported rates of adverse events remain highly variable. While one meta-analysis [166] associated CIW with a complication rate of 42.7%, a recent study [167] identified similar complication rates among patients with and without CIW wafers, even when combined with chemoradiation.
Interstitial chemotherapy is also limited by restricted distribution and rapid clearance of the free drug within and from the brain. Initial preclinical studies [168] in rabbits were promising, demonstrating that 3 days after wafer implantation, the effective depth of penetration of BCNU (defined as the distance from the polymer at which the concentration was 10% of its maximum value) was 10–12 mm. Given the evidence that the majority of HGG recurrences occur within 2 cm of the initial resection cavity, these data were felt to be encouraging. The limited distribution of BCNU is likely due to a combination of factors, including high interstitial pressure compared to pressure within the tumor resection cavity, the narrow width and high tortuosity of the brain ECS, and rapid clearance or partitioning into nearby cells. Nevertheless, experiments in non-human primates demonstrated high doses of BCNU (in the millimolar range) 3 mm from the wafer, as well as significant doses (in the micromolar range) throughout the entire brain. Importantly, 7 days following implantation, significant doses of BCNU persisted 1–2 cm from the wafer [169]. Postoperative edema may contribute to the convective flow of BCNU, allowing greater distribution than with diffusion alone. Additionally, while the lipophilicity of the drug contributes to rapid partitioning and/or clearance, it is possible that this same property allows BCNU to circulate within the blood and/or CSF and reenter the brain parenchyma at sites distant from the wafer [170].
Future Directions
Although CIW is the only local treatment approved for use in humans, a variety of therapeutic agents are being incorporated into polymer wafers and hydrogels for preclinical testing, including methotrexate [171], doxorubicin [171], carboplatin [172], 5-fluorouracil [173], ardipusilloside I [174], and butylidenephthalide [175, 176]. Additionally, biomaterial advances have resulted in improved polymers, new wafer designs, and most recently, implantable microchips consisting of multiple, discrete, drug-containing reservoirs [177]. Microchips enable controlled release via two mechanisms. On the one hand, each reservoir can be sealed with a polymer membrane; the composition and molecular mass of the polymer determine its rate of degradation, thereby allowing the contents of that reservoir to be released at a predefined point in time [178]. Alternatively, a metallic membrane can be incorporated and subsequently disintegrated by electrothermal ablation [179]. A resorbable polymer microchip containing BCNU and a microelectromechanical (MEMS) microchip containing temozolomide have both demonstrated efficacy in a 9L rat gliosarcoma model [180, 181], and the first clinical trial of an implantable microchip for drug delivery (for the treatment of osteoporosis) was recently completed [182]. Incorporating microchip technology into the treatment of malignant brain tumors would potentially allow more precise temporal control over the local delivery of chemotherapeutics.
Convection-Enhanced Delivery
CED: The Clinical Experience
A second strategy for interstitial delivery involves infusing a therapeutic agent directly into the region of interest via one or multiple stereotactically placed catheters. In 1994, Bobo et al. [183] described the underlying principles of CED—the continuous infusion of a therapeutic agent under pressure generates bulk flow. As a result, the distribution of the infusate is pressure driven, in contrast to therapeutic agents that are delivered via interstitial wafers or bolus injections, where the volume of distribution (V d) is dictated by diffusion—a process dependent on the concentration gradient. Whereas interstitial wafers result in a high concentration of drug in the immediately adjacent region followed by a steep drop-off, CED has been promoted for the ability to generate more constant concentrations over larger distances. Nevertheless, the experience with CED has shown that a number of factors influence the V d, including the infusion volume (V i), infusion flow rate, tissue parameters (e.g., the size of the ECS), and infusate parameters (e.g., molecular size and charge) [184].
Numerous phase I and phase II clinical trials have explored the safety and efficacy of CED for the delivery of various compounds. The ideal agent does not cross the BBB easily (thereby reducing its rate of clearance from the brain), specifically targets neoplastic cells, and is associated with non-CNS toxicities that preclude its systemic administration. Conventional chemotherapeutic agents that fit this description include topotecan and carboplatin, and preliminary phase I studies [185–187] suggest that CED allows these agents to be delivered at therapeutic concentrations while avoiding toxicity. The vast majority of studies, however, have explored the use of CED for the delivery of more exotic therapies that target a variety of tumor-specific antigens. These include antisense oligonucleotides that inhibit TGF-β2 [188], immunostimulating oligodeoxynucleotides containing CpG motifs [189], liposomal gene therapy vectors [190], and monoclonal antibodies conjugated to radioactive isotopes [191].
Targeted immunotoxins represent the most heavily studied class of compounds delivered via CED. Toxins in current use are obtained from either Corynebacterium diphtheria or Pseudomonas aeruginosa and modified by replacing the toxin’s native targeting mechanisms with tumor-specific ligands that recognize the transferrin receptor, interleukin-4 (IL-4) receptor, IL-13 receptor, or EGFR. Phase I and II studies have included TP-38 (Pseudomonas exotoxin fused with EGFR) [192], NBI-3001 (Pseudomonas exotoxin linked to IL-4) [193, 194], and TF-CRM107 (diphtheria toxin fused to transferrin C) [195, 196]. TF-CRM107, in particular, demonstrated a 50% decrease in tumor volume in 9 of 15 patients in an initial phase I trial; less impressive results in a subsequent phase II trial, however, resulted in the termination of a planned phase III trial.
As a result, the only CED agent to reach a phase III study has been cintredekin besudotox (CB), a Pseudomonas toxin fused with recombinant human IL-13. Phase I studies [197, 198] initially identified maximum tolerate infusate concentrations of 0.5 µg/mL, delivered via CED for 96 h. These parameters were then tested in the PRECISE trial [199], a phase III study in which patients were randomized 2:1 to receive CB versus CIW. The trial, which recruited 296 patients from 52 centers, was unfortunately deemed a failure, as it did not result in a statistically significant difference in median overall survival between the groups. Of note, there was a significant improvement in progression-free survival (17.7 vs. 11.4 weeks, p = 0.0008), but this was not a prespecified end point of the study. While trying to explain the negative outcome of the trial, some have pointed to flaws in its statistical design, while others have emphasized a subsequent analysis showing that, on average, the “coverage volume” included only 20% of the 2-cm rim around the resection cavity [200]. Suboptimal catheter positioning (only 51% of the catheters were accurately positioned) and the inability to accurately track the drug distribution in real time likely contributed to the poor distribution of CB [201].
Limitations of CED
The outcome of the PRECISE trial highlights some of the inherent limitations of CED in its current form. In the absence of real-time imaging to track the distribution of infusate, numerous factors can alter the geometry and V d in unpredictable ways. For example, leakage into the subarachnoid space and/or the ventricles can result in diminished concentrations at the region of interest, as well as a higher prevalence of toxicities, including chemical meningitis. Optimizing catheter placement is therefore critical in ensuring adequate distribution. Additionally, even when catheter positioning is appropriate, the tissue characteristics in and adjacent to a tumor are often heterogeneous and difficult to predict. Of note, the tumor is often characterized by a higher interstitial pressure than the surrounding tissue [202]; the pressure gradient therefore shunts the infusate away from the tumor.
Another process that reduces the effective V d involves “backflow” or “reflux” along the catheter. Preclinical studies [203] have shown that with larger cannula size, there is more trauma to the tissue upon insertion of the catheter. If the surrounding tissue is not tightly sealed around the catheter, the infusate will travel along the path of least resistance—along the catheter itself, instead of into the tissue. Similarly, high infusion rates promote reflux, although conversely, infusion rates that are too low allow the infusate to be cleared before adequate concentrations can accumulate. Optimizing the rate of infusion often requires finding a balance between the two extremes [204].
Future Directions
Since the early CED trials, a number of advances in the realms of engineering, mathematical modeling, and imaging have dramatically changed the landscape of tumor treatment with CED. Of note, CED was initially performed with simple, one-port catheters. However, a variety of more advanced catheters are now available. Reflux-free catheters involve different variations of a “stepped” cannula design, which allows for a small catheter tip while maintaining ease of placement [205, 206]. Multiple port catheters are characterized by multiple openings, although studies have shown that the infusate is, in reality, only delivered from the most proximal port [184]. Porous hollow fiber catheters have walls that consist of millions of pores with diameters of 0.45 µm in order to increase the surface area exposed to the infusate [207]. Balloon-tipped catheters incorporate a balloon proximal to the tip of the catheter, which can be expanded to fill the resection cavity and prevent reflux during the infusion. Early results in a canine model are promising [208].
In addition to newer catheter designs, being able to predict the distribution of the infusate ahead of time would have significant implications for planning catheter placement and infusion volumes. Rigorous mathematical modeling and computational methods have begun to account for the anisotropy and heterogeneity of the human brain, particularly in the setting of tumor infiltration [209–212]. Until such models are perfected, however, the only way to know the spatial distribution of the infusate is through real-time imaging. MRI has been used to monitor the distribution of liposomes delivered via CED by incorporating gadolinium–diethylene triamine pentaacetic acid (Gd-DTPA) into the liposome construct [213]. Alternatively, Gd-DTPA can be coinfused with the therapeutic agent during CED [214]. For those who would prefer to visualize the therapeutic agent itself, iron oxide nanoparticles can be imaged directly with MRI, thus enabling real-time adjustments to the flow rate if reflux occurs [215].
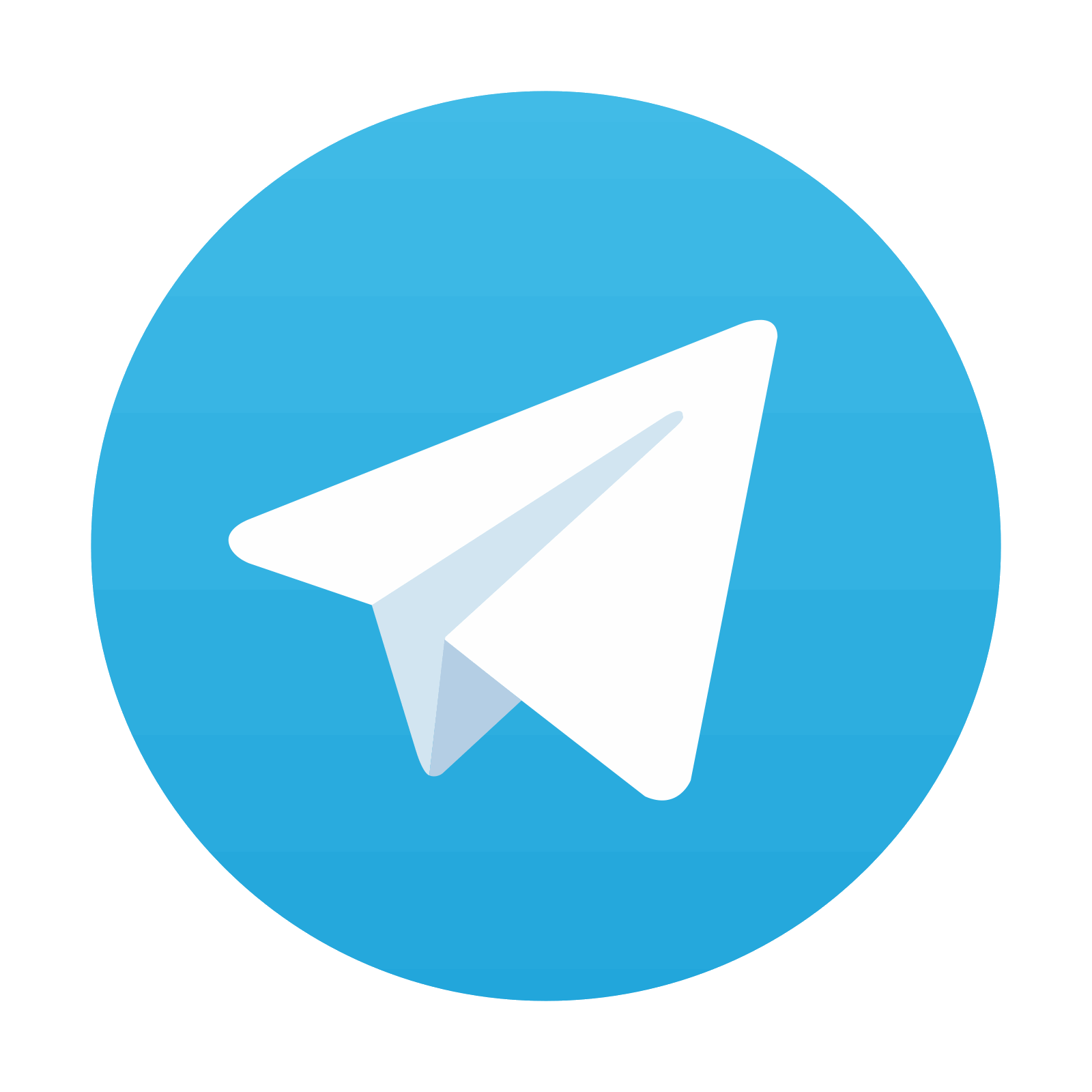
Stay updated, free articles. Join our Telegram channel
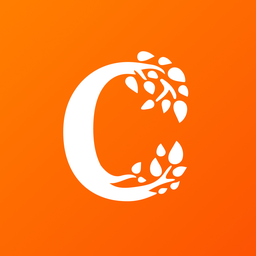
Full access? Get Clinical Tree
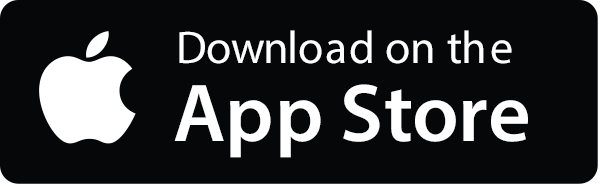
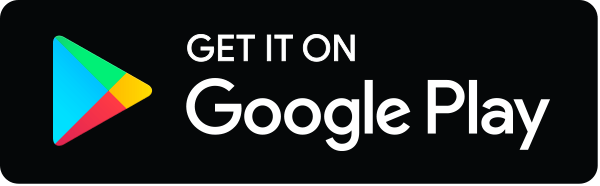
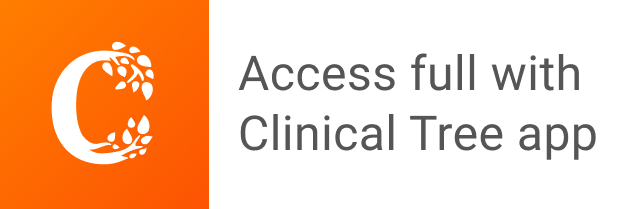