Fig. 1
The thalamocortical loop. The red curved arrow symbolizes the intra-cortical processing of sensory information. Note that layer 6 pyramidal cell projects on the distal part of the thalamic dendrite (Color figure online)
1.1.1 The Corticothalamocortical Loop
The organization of the thalamocortical loop is roughly similar for all sensory thalamic nuclei and their cortical counterparts (Fig. 1). Relay cells of the sensory thalamus send glutamatergic projections to the neocortex and to the thalamic reticular nucleus (TRN). In rodent species, TRN cells provide the only source of inhibitory inputs to the sensory nuclei (Jones 2007). In the cortex, relay cells project mainly on layer 4 neurons, while corticothalamic feedback is provided by a subpopulation of layer 6a pyramidal neurons. In contrast to sensory inputs, which arrive on the proximal part of the thalamic dendrite, layer 6a neurons target the distal part of the relay cells. These corticothalamic returns contribute to almost 50 % of the distal synapses on thalamic relay cells and provide a powerful tool for the cortex to regulate thalamic activity (Sherman and Guillery 1996; Erisir and Sherman 1997; Varela 2014). However, this dense synaptic network is balanced by the small and long-lasting responses elicited by cortical inputs which are due to the weak activation of ionotropic receptors and to the reliable activation of metabotropic receptors (Miyata and Imoto 2006; Turner and Salt 1998). In contrast, thalamic inputs trigger a strong time-scaled response in the cortex, consistent with their role in the transfer of sensory information (Swadlow 2003; Cruikshank et al. 2007). These reciprocal interactions play a central role in shaping thalamocortical oscillations during deep sleep, and may be involved in the sleep-wake transition.
1.1.2 Thalamocortical Dynamics Across Sleep-Wake Cycle
Oscillations measured by electroencephalogram (EEG) at different stages of a sleep-wake cycle reflect thalamocortical loop dynamics. Indeed, deep sleep is characterized by slow and ample oscillations of the EEG resulting from the synchronization of thalamic and cortical networks while EEG of awake animals exhibits rapid and short oscillations characteristic of desynchronized networks.
Slow and ample oscillations are the main EEG hallmarks of deep sleep. These oscillations are quasi-synchronous across cortical areas and involve virtually all the cortical neurons. Although both cortical and thalamic networks in isolation are able to trigger slow-wave like oscillations in certain conditions, the constant communication between both networks through the thalamocortical loop is essential to produce physiological slow oscillations (Steriade et al. 1993; David et al. 2013). For instance, thalamic neurons require the activation of distal mGluRs receptors by corticothalamic inputs to generate oscillations, whereas cortical oscillations are fine-tuned by thalamic oscillations to reach physiological frequencies (Crunelli and Hughes 2010; Gutierrez et al. 2013; David et al. 2013).
The frequency of slow cortical oscillations varies across sleep stages according to the bursting frequency of thalamic neurons (Crunelli and Hughes 2010). This bursting frequency is mainly shaped by the hyperpolarization of the resting membrane potential. Indeed, the duration of a thalamic burst depends on the aperture of T-type channels, which trigger a low-threshold Ca2+ current crowned by Na+ action potentials (Contreras and Steriade 1995). These T-type channels are inactivated for depolarized membrane potentials and deinactivated by membrane hyperpolarization, meaning that the percentage of T-type Ca2+ channel putatively activated is correlated to the membrane hyperpolarization. Hyperpolarization is due to the aperture of a K+ leak conductance, sustained by several types of channels, among them the TWISK-related acid-sensitive K+-channel (Task1 and Task3) (Meuth et al. 2006). K+ conductances are of primary importance since orexin affects membrane dynamics primarily by closure of K+ channels, meaning that orexin may directly impact the waveform of the slow oscillations of the thalamus.
1.2 Non-specific Thalamic Nuclei: Relays of Ascending Reticular Activating System and Dedicated Roles
1.2.1 Non-specific Thalamic Nuclei as Broad Cortical Activators
Whereas the role and organization of specific thalamic nuclei have been well characterized, non-specific thalamic nuclei have long been neglected and only few studies aim at dissecting the precise role of these nuclei in the maintenance of arousal states. Because of their widespread projections to the neocortex, midline and intrathalamic nuclei, the two main sets of non-specific thalamic nuclei, have long been considered as global cortical activators (Lorente de No 1938; Herkenham 1980; Steriade and Glenn 1982). This hypothesis is supported by the pioneering experiments of Dempsey and Morison showing that electrical stimulation of midline and intralaminar thalamic nuclei is able to induce a broad activation of the cortical network (Morison et al. 1941; Dempsey et al. 1941; Morison and Dempsey 1942; Dempsey and Morison 1942). Subsequent experiments by Moruzzi and Magoun demonstrate that non-specific thalamic nuclei relay information from the ascending brainstem arousal system to the cortical areas and is thus involved in the promotion of cortical arousal (Moruzzi and Magoun 1949; Steriade and Glenn 1982).
Clinical evidence supports this hypothesis. Large lesions of the non-specific thalamic nuclei induced by strokes or by the alcoholic Korsakoff syndrome have been shown to affect the consciousness of patients (Schmahmann 2003; Carrera and Bogousslavsky 2006). Moreover, injuries of the midline thalamic nuclei, which are the nuclei that receive the densest projections from orexin neurons, lead to major deficits in arousal and memory (Schmahmann 2003). In contrast, lesions disrupting other thalamic nuclei affect primarily mood and lead to disinhibited behaviors (Schmahmann 2003). Brain imaging indicates that the spatial extent of the thalamic lesion is correlated to the severity of the syndromes. Partial lesions impact attention, which often trigger a deficit in working and long-term memory, while entire lesions of the median thalamic nuclei are associated with a state close to coma (Façon et al. 1958; Castaigne et al. 1962; Tinuper et al. 1989; Llinas et al. 1998; Carrera and Bogousslavsky 2006). Moreover, stimulation of non-specific thalamic nuclei in patient suffering from minimal conscious state has been shown to improve their symptoms, indicating that these nuclei can promote cortical arousal (Schiff et al. 2007).
Evidence from physiological experiments explicits the activity of non-specific thalamic nuclei across sleep-wake cycle. In rodents, electrical activity and neuronal excitability increase during the dark period, which corresponds to their active period (Novak and Nunez 1998; Steriade and Glenn 1982). Electrical stimulation of these non-specific thalamic nuclei produces a broad activation of cortical networks, that is likely to drive a tonic state of readiness in cortical networks, allowing performing high-demanding tasks (Glenn et al. 1982; Steriade and Glenn 1982). Moreover, high frequency stimulation of non-specific thalamic nuclei is sufficient to shift the cortical dynamics from slow and ample oscillations to fast awake-like activity (Steriade and Llinas 1988; Steriade 1997). Recordings of human brain activity using positron emission topography indicate that besides being involved in the sleep to wake transition, non-specific nuclei are also implicated in the transition between relaxed wake and high general attention (Kinomura et al. 1996). This tonic activation of cortical networks is driven by the widespread projections of non-specific thalamic nuclei to layers 1, 5 and 6, which contain the majority of neurons activated in attentive behaviors (Hyvarinen et al. 1980; Herkenham 1980; Glenn et al. 1982; Berendse and Groenewegen 1991; Clasca et al. 2012; Cruikshank et al. 2012).
1.2.2 Non-specific Thalamic Nuclei and Their Dedicated Roles
Refinement of anatomical tracing as well as behavioral and functional studies however indicate that non-specific thalamic nuclei take part in more dedicated tasks. Each nucleus sends projections to a small number of associative brain regions, among them the striatum, hippocampus, amygdala and several cortical areas, to provide information about the environmental and behavioral context (Jones and Leavitt 1974; Macchi et al. 1975, 1977; Berendse and Groenewegen 1991; Groenewegen and Berendse 1994; Giménez-Amaya et al. 1995; Van der Werf et al. 2002; Vertes et al. 2006). Furthermore, functional studies indicate a preferential activation of certain non-specific thalamic nuclei according to the task performed by the animal (Deutch et al. 1995; Vann et al. 2000; Loureiro et al. 2012; Mitchell and Chakraborty 2013; Xu and Südhof 2013).
Among them, the rhomboid and reuniens thalamic nuclei, two nuclei belonging to the midline thalamic nuclei, are activated during goal-directed tasks that require a high level of attention (Hembrook and Mair 2011; Hembrook et al. 2012; Loureiro et al. 2012; Cholvin et al. 2013). Due to the privileged position of rhomboid and reuniens nuclei at the interface between prefrontal cortex and hippocampus, they are thought to orchestrate the communication between these regions during high-demanding tasks (Cholvin et al. 2013; Cassel et al. 2013).
As for the other non-specific thalamic nuclei, they have been involved in tasks as diverse as stress response, spatial navigation, drug addiction or head direction coding. Therefore, thalamic nuclei have dedicated roles that all require increased attention and contribute all together to the maintenance of states of arousal.
In conclusion, though acting at different steps of the sleep-wake cycle, both thalamic pathways are involved in the regulation of cortical states of arousal. Specific thalamic nuclei take part in the maintenance and shaping of slow-wave oscillations occurring during deep sleep, while activation of non-specific thalamic nuclei is associated with sleep-wake transitions and enhancement of cortical arousal. Thalamic pathways are differentially impacted by upper brainstem inputs, and interestingly they are differentially targeted by orexin neurons.
2 Projections of Orexin Neurons on Thalamic Nuclei and Cortical Areas
Orexin neurons of the lateral hypothalamus project widely throughout the brain with densest projections in the regions involved in arousal and attention.
2.1 Projections on Non-specific Thalamic Nuclei
Labeling of orexin fibers using antibodies against the two orexin proteins, orexin-A and orexin-B, or their common precursor, the prepro-orexin, reveal a heterogenous pattern of projection throughout the thalamus (Peyron et al. 1998; Chen et al. 1999; Nambu et al. 1999; Date et al. 1999; Cutler et al. 1999; Mintz et al. 2001; Kirouac et al. 2005). Orexin fibers project widely to non-specific thalamic nuclei while they poorly target specific nuclei. Orexin neurons projecting to the thalamus are distributed within the hypothalamus with a highest density in the perifornical nucleus and in the ventral part of the lateral hypothalamus (Kirouac et al. 2005; Lee 2005). Axons exit the hypothalamus by the ascending pathway and project throughout the majority of the intralaminar and median thalamic nuclei following a dorsolateral to ventromedial orientation (Peyron et al. 1998). They enter the thalamus through the zona incerta, which provides GABAergic inputs to higher order thalamic nuclei, and ramify in this structure, indicating that inhibitory neurons of the thalamus are strongly modulated by orexin (Peyron et al. 1998; McGranaghan and Piggins 2001; Jones 2007). Orexin fibers target primarily the paraventricular nucleus and to a lesser extent the central medial, rhomboid, reuniens, intramediodorsal, centrolateral and lateral habenular nuclei (Peyron et al. 1998; Nambu et al. 1999; Date et al. 1999; Mintz et al. 2001; Kirouac et al. 2005; Hsu and Price 2009). In these nuclei, orexin axons can exhibit a smooth or a varicose morphology, suggesting the coexistence of synaptic and volume transmission. The colocalization of orexin and synaptophysin, a protein present in synaptic buttons, supports the hypothesis of a synaptic release of orexin in non-specific nuclei, in agreement with electron microscopy analysis in other brain areas (Peyron et al. 1998; Kirouac et al. 2005). In addition to this point-to-point release in the thalamus, some orexin fibers crossing the thalamic nuclei are thought to release orexin in the dorsal third ventricle. These fibers have been reported in the ventricular wall at the boundary of the paraventricular nucleus and may contribute to a global effect of orexin in the brains (Mintz et al. 2001).
In contrast, orexin fibers are very sparse in sensory nuclei, except in the intergeniculate leaflet (IGL). The IGL belongs to the lateral geniculate nucleus, which primarily relays visual information from the retina to visual cortices (Peyron et al. 1998; Mintz et al. 2001). In contrast to most subnuclei of the lateral geniculate nucleus, the IGL is not only a relay of primary visual information but is also an important component of the circadian regulator system. Indeed, it is involved in transferring photic and non-photic cues from the retina to the suprachiasmatic nucleus, which in mammals is the main circadian oscillator (Reppert and Weaver 2002). The IGL has also been involved in the transfer of non-specific information to the suprachiasmatic nucleus, especially information from structures involved in the sleep-wake cycle. Thus, the specific innervation of the IGL by orexin fibers is consistent with their common involvement in sleep-wake cycle regulation.
Interestingly, alongside a high homology of orexin sequences, the differential targeting of thalamic nuclei is highly conserved among vertebrates (Alvarez and Sutcliffe 2002). Orexin fibers are restricted to thalamic areas that project to the limbic system in both diurnal and nocturnal mammals (Mintz et al. 2001; McGranaghan and Piggins 2001), birds (Singletary et al. 2006), amphibians (Shibahara et al. 1999; Lopez et al. 2009a; Suzuki et al. 2007) and fish (Huesa et al. 2005; Lopez et al. 2009b). The conservation of the projection pattern of orexin neurons contrasts with the divergence observed for other neuropeptides (Mintz et al. 2001), suggesting that the innervation of non-specific thalamic nuclei by orexin neurons plays a conserved role in animal physiology.
Measure of orexin concentration in brain tissues reveals an equivalent release of orexin in the paraventricular thalamic nucleus, the lateral hypothalamus and the locus coeruleus (Mondal et al. 1999b). Accordingly, the density of post-synaptic buttons expressing orexin receptors is comparable in the locus coeruleus and in the paraventricular nucleus (Huang et al. 2006). The strong modulation locus coeruleus dynamics by orexin has been assessed in the literature at the cellular and system levels, as reported in other chapters of this book (Carter et al. 2009, 2012). This first confirms that non-specific thalamic nuclei are one of the major target for orexin neurons and second suggests that orexin play a strong and under-estimated role on the modulation of thalamic dynamics. Orexin-A and orexin-B positive fibers largely overlap in the thalamic nuclei, suggesting that most orexin fibers release both peptides (Date et al. 1999; Zhang et al. 2002, 2004). However, local disparities have been reported throughout the brains which point out the differential involvement of these peptides in the modulation of physiological modalities. In the thalamus, orexin-B antibody labels thinner and sparser axons in most nuclei and only orexin-A positive fibers are found to reach the ventricular wall at the border of the paraventricular nucleus (Cutler et al. 1999; Zhang et al. 2002, 2004). In contrast to these labelings, measures of orexin concentration reveal a three times higher concentration of orexin-B compared to orexin-A in thalamic tissues (Mondal et al. 1999a). Conversely, in the locus coeruleus, labelings and measure of orexin concentrations are consistent and indicate a predominant release of orexin-A. These contrasting results indicate first a differential release of orexin-A and -B according to the cerebral structure and second suggest that orexin-B plays a primary role in the modulation of orexin-dependent processes in thalamic neurons.
The sensibility to orexin is conferred by post-synaptic expression of G-coupled receptors for orexin, which present two isoforms called OX1R and OX2R (Sakurai et al. 1998). OX1R exhibits a preferential affinity for orexin-A while OX2R affinity is equivalent for both orexin peptides. Distribution of OX1R and OX2R throughout the thalamus has been extensively studied using in situ hybridization. It shows that orexin receptors are mainly expressed by midline and intralaminar thalamic nuclei, in agreement with the projection profile of orexin fibers (Trivedi et al. 1998; Lu et al. 2000; Marcus et al. 2001). In contrast, orexin receptors are barely detected in the specific thalamic nuclei. Within non-specific thalamic nuclei, OX1R and OX2R are distributed according to a precise pattern. Expression of mRNAs coding for OX1R is restricted to paraventricular neurons and is present to a lesser extent in the reuniens and parafascicular nuclei (Trivedi et al. 1998; Lu et al. 2000). In contrast, mRNAs coding for OX2R are detected in most intralaminar and midline thalamic nuclei, the highest density being noticed in the central median, rhomboid, paraventricular and centrolateral nuclei (Trivedi et al. 1998; Lu et al. 2000; Marcus et al. 2001). At the protein level, OXRs are observed widely throughout the brain and especially in the thalamus, suggesting that OXR expression was underestimated by in situ hybridization experiments (Hervieu et al. 2001; Cluderay et al. 2002). However, the strength of the labeling varies between regions and consistently with mRNA detection, the strongest labelings are detected in the central median, rhomboid, paraventricular, parafascicular, reuniens and centrolateral nuclei.
Altogether, these results indicate that non-specific thalamic nuclei are privileged targets for orexin modulation in contrast to specific nuclei that are poorly targeted by orexin fibers. Labelings show an overlap of the OXR expression and orexin fibers in most non-specific thalamic nuclei, with a highest density of fibers and receptors measured in the paraventricular, centromedian, rhomboid and reuniens nuclei. Furthermore, orexin-B is primarily involved in non-specific thalamic modulation, in agreement with the preferential expression of OX2R in these nuclei.
2.2 Projections on Cortical Areas
Orexin neurons send sparse projections to the neocortical areas through the dorsal ascending pathway (Peyron et al. 1998). These afferences, especially those projecting to the medial prefrontal cortex, come from a small subset of neurons distributed within the entire lateral hypothalamus (Fadel et al. 2002). Labelings using either anti-prepro-orexin, anti-orexin-A or anti-orexin-B antibodies reveal a substantial targeting of associative cortical areas whereas sensory cortices receive only sparse projections. Among associative areas, the retrosplenial and prefrontal cortices, especially the insular, prelimbic and infralimbic cortices and to a lesser extent the perirhinal and piriform cortices exhibit the highest density of fibers (Peyron et al. 1998; Date et al. 1999; Nambu et al. 1999; Cutler et al. 1999). Interestingly, neocortical regions exhibiting the highest density of orexin fibers belong to the associative areas, that processes higher-order information, highly dependent of arousal states. These labelings indicate that orexin differentially modulates cortical areas according to the nature of information processed.
Across cortical layers, orexin fibers are primarily located in layer 6 and to a lesser extent in layer 1 (Peyron et al. 1998; Nambu et al. 1999). Orexin fibers are oriented radially from the white matter to the pial surface, except in layer 6 where they run along the white matter towards the corpus callosum (Peyron et al. 1998; Nambu et al. 1999). Cortically projecting fibers exhibit both smooth and varicose morphology, as observed in the thalamus (Cutler et al. 1999). The concentration of orexins measured in cortical tissues is roughly equivalent for orexin-A and -B, in agreement with the global overlapping of orexin-A and -B positive fibers in the cortex (Date et al. 1999; Mondal et al. 1999a). However, this concentration is ten times lower than those measured in the thalamus, probably due to the sparse projections of orexin fibers in the neocortex (Mondal et al. 1999a). It is noteworthy that this concentration has been measured within the entire cortical column and may not reflect the real concentration in the layer 6, which receives the majority of the orexin fibers. Refinement of this measure would thus be important to better understand the physiological impact of orexin in the deep layers of cortical areas.
Expression of orexin receptors is weaker in the neocortex than in the non-specific thalamus and displays high heterogeneity (Trivedi et al. 1998; Hervieu et al. 2001; Cluderay et al. 2002). OX2R is strongly expressed in the deep layers whereas a diffuse expression of OX1R and OX2R mRNAs is detected throughout the rest of the cortical layers (Trivedi et al. 1998; Lu et al. 2000). Refinement of this observation using single-cell RT-PCR indicates that the strong expression of OX2R in the deep layers is restricted to layer 6b in the somatosensory cortices (Hay et al. 2014). In the infralimbic, cingulate, dorsal peduncular and granular insular regions of the prefrontal cortex, layers 2, 5 and 6 neurons express a relatively high level of OX1R mRNA compared to the very weak detection of OX1R in other cortical areas (Marcus et al. 2001). Accordingly, protein level of OX1R is higher in the frontal cortex than in somatosensory cortices (Aracri et al. 2013). Furthermore, layer 5 neurons of the somatosensory cortex exhibit a low labeling, although in some cases a clear pyramidal morphology can be observed. In contrast, layer 5 cells of the frontal cortex exhibit a dense labeling of the soma and some punctiform labeling of the neuropil (Hervieu et al. 2001; Aracri et al. 2013). Labeling of OX2R protein confirms the strong expression of this receptor in layer 6b neurons but also indicates a substantial expression by pyramidal cells of the prefrontal cortex (Cluderay et al. 2002).
Taken together, these anatomical data indicate a heterogenous distribution of orexin fibers and orexin receptors throughout cortical areas. This suggests that orexin impacts strongly and globally the dynamics of the associative cortical areas whereas sensory cortical networks are precisely modulated through the activation of layer 6b neurons. Furthermore, in associative cortices activation of both OX1R and OX2R participate to the modulation of cortical dynamics whereas in sensory cortices OX2R only is implicated.
3 Orexin Regulates Thalamocortical Pathway by Pre- and Post-synaptic Mechanisms
3.1 Somatodendritic Activation of Thalamic and Cortical Neurons by Orexin
Electrophysiological recordings in rodent brains slices allow describing precisely the electrical responses to orexin application. In thalamic and cortical neurons, orexin application induces a long-lasting depolarization of the cells peaking 2–4 min after the onset of the application. Suprathreshold depolarizations are crowned by a long train of tonic action potentials or by a sustained train of bursts (Bayer et al. 2002, 2004; Kolaj et al. 2007; Hay et al. 2014; Govindaiah and Cox 2006; Ishibashi et al. 2005). Upon washing, the response decreases slowly and gradually, until returning to the initial state after 10–15 min (Kolaj et al. 2007). Similar profile of responses are obtained upon application of orexin-A or -B although the amplitude of response varies across regions according to the relative expression of each OXR.
Proportion of orexin-sensitive neurons varies across areas according to the density of orexin fibers while the response magnitude to either orexin-A or -B in each area is correlated to the local expression of OXRs. In the thalamus, virtually all paraventricular, central median and rhomboid neurons are depolarized by orexins, whereas responses are detected in only 70 % neurons of the centrolateral nucleus and even less in the mediodorsal and reticularis nuclei (Bayer et al. 2002; Govindaiah and Cox 2006; Kolaj et al. 2007; Ishibashi et al. 2005; Huang et al. 2006). Orexin-A and -B trigger similar responses in most non-specific thalamic neurons, indicating that the sensitivity to orexin is likely to be mediated by OX2R. In contrast, in the reticularis neurons, orexin-A drives a stronger depolarization than orexin-B, indicating that the depolarization is likely to be mediated by OX1R activation (Govindaiah and Cox 2006). We mentioned previously that specific thalamic nuclei are free of orexin fibers and display few or no expression of OXRs. Consistently application of orexin has no effect on the resting membrane potential or on the membrane resistance of these neurons (Bayer et al. 2002; Govindaiah and Cox 2006).
In most cortical areas, neurons sensitive to orexin are located in layer 6b, in agreement with the exclusive expression of OX2R in deep layers and with the sparse density of orexin fibers throughout the cortical layers except in deep layers (Trivedi et al. 1998; Peyron et al. 1998; Bayer et al. 2004; Hay et al. 2014). Interestingly, both excitatory and inhibitory neurons of layer 6b are activated by orexin (Hay et al. 2014). This restricted sensitivity of cortical neurons to orexin is consistent with the low occurrence of orexin-induced response in primary culture of cortical cells (Pol et al. 1998). In prefrontal cortices, which exhibit the highest density of orexin fibers in the neocortex, the situation remains to be clarified as orexin sensitivity has been reported in layer 5 pyramids and to a lesser extend in layer 2 pyramids by some groups (Xia et al. 2005; Song et al. 2005; Li et al. 2010), whereas others mention restricted responses to layer 6b neurons at least in the cingulate cortex (Bayer et al. 2004). One may suggest that this difference is due to the fact that Bayer and colleague. have used preferentially orexin-B for their experiments, which would have made the OX1R-mediated response undetectable. Furthermore, the highest density of orexin fibers in the prefrontal cortex is consistent with a broader responsivity across cortical layers, and OX1R labeling has been observed in layer 2 and 5 pyramidal cells (Li et al. 2010; Aracri et al. 2013).
3.2 Mechanisms of Orexin-Induced Somatodendritic Activation
The amplitude of orexin-mediated depolarization in both thalamic and cortical neurons depends on orexin concentration. For neurons expressing mainly OX2R, i.e. neocortical layer 6b and non-specific thalamic neurons, orexin-B is slightly more potent for low doses of orexin while higher doses lead to similar amplitude of depolarization in response to orexin-A and -B. Indeed, a subset of these neurons exhibit responses to orexin-B from 10 nM while no response to orexin-A is observed at this concentration. Furthermore, EC50 is reached at a concentration of 20 nM for orexin-B and 32 nM for orexin-A. At higher concentration (1000 nM), both peptides trigger a large depolarization crowned with a massive discharge of action potentials (Bayer et al. 2002, 2004; Ishibashi et al. 2005; Govindaiah and Cox 2006; Huang et al. 2006). While the EC50 for orexin-A is consistent with previous report using human isoform of the OXRs, the EC50 for rodent orexin-B is at least two times lower than those previously described (Sakurai et al. 1998; Beuckmann and Yanagisawa 2002). That suggests that the EC50 measured for orexin-B in heterologous system may have been underestimated and as a consequence that OX2R would be more sensitive for orexin-B than for orexin-A.
Direct somatodendritic activation of thalamic and cortical neurons is assessed by the persistence of orexin responses in presence of TTX (Bayer et al. 2002, 2004; Ishibashi et al. 2005; Huang et al. 2006; Govindaiah and Cox 2006; Kolaj et al. 2007; Li et al. 2010). Indeed, TTX, by acting on Na+ voltage-dependent channels, prevents propagation of action potentials, blocks synaptic transmission and as a consequence isolates neurons from each other. Furthermore, orexin effects persist in the presence of glutamatergic and GABAergic inhibitors confirming a direct action of orexin on the somatodendritic compartment instead of an indirect network-mediated effect (Huang et al. 2006). A final argument to assess the post-synaptic activation of non-specific thalamic and layer 6b cortical neurons is provided by the enhancement of the orexin response in low-Ca2+ and high-Mg2+ concentration conditions. Indeed, in these conditions, the release probably decreases dramatically, which blocks the synaptic transmission (Bayer et al. 2002, 2004; Ishibashi et al. 2005; Huang et al. 2006). Thus, several pieces of evidence indicate that, in non-specific thalamic nuclei as well as in cortical layer 6b, orexin acts on neurons by a direct somatodendritic activation.
Most orexin-mediated depolarizations are coupled with an increase of the membrane resistance, signaling channel closure. This increase of membrane resistance reaches a maximum of 150 % in the paraventricular neurons and is milder in other non-specific thalamic nuclei and in layer 6b neurons (Ishibashi et al. 2005; Bayer et al. 2002, 2004; Govindaiah and Cox 2006; Huang et al. 2006; Doroshenko and Renaud 2009; Kolaj et al. 2007). Information about the ion involved in this conductance is provided by plotting the current-voltage relationship (I–V), which reveals that the orexin-induced current reverses at the equilibrium potential of K+. Moreover the I–V curve is right-shifted in the presence of an increased concentration of external K+, pointing out that orexin-mediated current is due to the closure of a K+ conductance (Govindaiah and Cox 2006; Bayer et al. 2002, 2004; Huang et al. 2006). Several K+-channels are expressed by thalamic and cortical cells. In the thalamus, it is noteworthy that Task1 and Task3 channels, which are involved in the hyperpolarization of thalamic cells during sleep, are also implicated in orexin-induced depolarization (Doroshenko and Renaud 2009).
Several other mechanisms have been implicated in orexin-induced depolarization, such as the aperture of a cationic non-specific conductance or the inactivation of a HCN channel (Huang et al. 2006; Kolaj et al. 2007; Li et al. 2009). Aperture of a conductance is confirmed by the increase of the background noise in the presence of orexin (Huang et al. 2006). This mechanism has been observed in some cells in layer 2 and 5 of the prefrontal cortex and in few non-specific thalamic neurons (Huang et al. 2006; Kolaj et al. 2007), whereas inactivation of HCN channels have been reported only in pyramidal cells of the prefrontal cortex upon activation of OX1R (Li et al. 2009).
3.3 Pre-synaptic Activation of Non-specific Thalamocortical Terminals in the Prefrontal Cortex
In addition to the somatodendritic activation of cortical neurons, orexin has been shown to act directly on a subset of pre-synaptic terminals in the prefrontal cortex (Lambe and Aghajanian 2003). Using a combination of patch-clamp recording and calcium imaging, Lambe and colleague have demonstrated that the application of orexin-B does not affect the resting membrane potential of layer 5 cells but selectively depolarize a limited number of dendritic spines. These local depolarizations are due the indirect activation of glutamatergic terminals, as assessed by the large increase of EPSC frequency in response to orexin-B application. A subsequent report shows that the increase of EPSC frequency persists in the presence of TTX, meaning that the orexin-induced EPSCs are due to the local depolarization of pre-synaptic glutamatergic terminals (Aracri et al. 2013). Non-specific thalamic fibers exhibit a close vicinity with the dendritic buttons depolarized during orexin application in the prefrontal cortex, suggesting that orexin activates specifically these thalamic terminals (Lambe and Aghajanian 2003). This hypothesis is supported by the absence of EPSC frequency increase when thalamic nuclei are lesioned (Lambe and Aghajanian 2003). On layer 5 pyramidal cells, orexin-activated buttons are primarily located on the apical tuft of the dendritic tree and to a lesser extent on the basilar dendrites, which is consistent with the projection pattern of non-specific thalamic nuclei (Berendse and Groenewegen 1991; Lambe and Aghajanian 2003). Thus, in addition to a direct post-synaptic activation of both cortical and thalamic neurons, non-specific thalamocortical pathway is also potentiated by orexin through the specific activation of pre-synaptic terminals.
However, the authors indicate that roughly 10 % of dendritic buttons are not apposed to non-specific thalamocortical fibers (Lambe and Aghajanian 2003). The discovery in 2004 of the specific sensitivity of layer 6b cells to orexin may provide a cue to explain the origin of these remaining buttons (Bayer et al. 2004; Lambe et al. 2007).
The presence of orexin receptors in pre-synaptic terminals was not investigated in other cortical areas, probably due to the weak effect of orexin on EPSC frequency compared to the strong one measured in prefrontal neurons (Lambe and Aghajanian 2003). Recently, the development of optogenetics tools that allow a specific photo-stimulation of non-specific thalamocortical pathways has permitted to address this question in the somatosensory cortex (Hay et al. 2014). Measure of the paired pulse ratio between two successive stimulations of the presynaptic terminals allow evaluating the pre-synaptic impact of a drug. Orexin does not impact this ratio at the synapse between non-specific thalamocortical fibers and cortical dendrites, indicating an absence of pre-synaptic modulation in the somatosensory cortex (Hay et al. 2014). Moreover, thalamocortical inputs recorded in layer 6a pyramidal cells are followed by a disynaptic current in the presence of orexin, which is prevented by the physical separation of layer 6b from the rest of the cortical layers (Hay et al. 2014). This indicates that in the somatosensory cortex, orexin acts selectively by post-synaptic activation of layer 6b neurons, as suggested by Lambe and Aghajanian (2003). Finally, analysis of the paired-pulse ratio in paraventricular thalamic neurons suggests as well an absence of pre-synaptic modulation by orexin within the thalamic nuclei (Kolaj et al. 2007).
As a consequence, in most non-specific thalamocortical pathways, orexin acts primarily through a post-synaptic activation of cortical and thalamic neurons. However, in certain cortical areas, such as the prefrontal cortex, orexin can also trigger presynaptic activation of non-specific thalamic terminals. It is noteworthy that the prefrontal cortex receives the strongest orexin inputs, acting via pre- and post-synaptic mechanisms, since prefrontal activity is highly correlated to the state of vigilance of the animal.
4 Orexin Modulates Vigilance States by Acting on the Thalamocortical System
We have shown that deep sleep is characterized by slow oscillations of the thalamocortical system while arousal is characterized by depolarization of the cells and desynchronization of the network. It is also known that sustained attention involves an increase of neuronal excitability and a synchronization of fast oscillations across cortical areas. Finally it has been reported that the induction of attention can be triggered by thalamic bursting. In this chapter, I will describe how orexin acts on these different parameters linked to the states of vigilance.
4.1 Modulation of Cortical Dynamics and Integration of Extra-Cortical Inputs by Orexin
4.1.1 Increase of Neuronal Excitability Throughout Cortical Layers in Primary Sensory Cortices
In the somatosensory cortex, activation of layer 6b neurons by orexin results in increased EPSC frequency primarily in infragranular layers and secondarily in layer 1 interneurons (Lambe and Aghajanian 2003; Bayer et al. 2004; Hay et al. 2014). This pattern is consistent with the projections of layer 6b in the cortical column and indicates that activation of layer 6b by orexin promotes an increase of cortical excitability (Clancy and Cauller 1999; Marx and Feldmeyer 2013). Besides this direct activation of the cortical network, orexin triggers action potential discharge in non-specific thalamic neurons by a direct somatodendritic mechanism, which may result in an increase of glutamatergic inputs in the cortex. Interestingly, layer 6b and non-specific thalamic neurons exhibit an overlapping projection pattern in the neocortex, since thalamic neurons also target primarily layers 1 and 5–6 (Herkenham 1980). This suggests that in most cortical areas, release of orexin activates two pathways running in parallel, leading to an increase of neuronal excitability specifically in layers 1 and 5–6. In layer 1, thalamocortical fibers target both layer 1 interneurons and the dendritic tuft of the pyramidal neurons (Krettek and Price 1977; Cruikshank et al. 2012; Clasca et al. 2012). This activation of apical dendrites has been hypothesized to promote a tonic state of readiness in cortical networks, typical of attentive states (Steriade and Morin 1981). Furthermore, this specific activation of cortical layers 1 and 6 is consistent with the observation that these layers contain the majority of neurons activated during attentive states, indicating a prominent role of these two cortical layers in the maintenance of attentive states (Hyvarinen et al. 1980).
In contrast, layer 4 cells are neither depolarized nor indirectly excited by orexin (Hay et al. 2014). This layer is the main target of specific thalamic nuclei and as a consequence receives mostly somatosensory inputs, whereas non-specific thalamic fibers only sparsely project to this layer. This suggests that in sensory cortices, sensory inputs are not directly influenced by cortical states of vigilance at their entrance to the neocortex (Fig. 2).
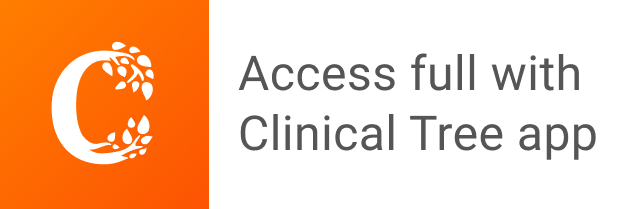