“Monitors themselves do not save lives, but the insightful interpretation of the information derived from them can.” —Saul and Ducker
Introduction
Traumatic brain injury (TBI) in the United States affects more than 1.5 million individuals per year costing more than $60 billion in both direct medical and indirect costs such as loss of productivity. Following the initial insult, a series of complex pathophysiologic cascades are set in place, which synergistically interact to cause delayed, secondary brain damage, over hours, weeks, and even up to a year after the TBI. Physicians have the capability to monitor and reverse these secondary damage mechanisms. A host of modalities, including cerebral blood flow (CBF), brain oxygen, intracranial pressure (ICP), biomarkers, and electrical cortical activity, among others, are available in state-of-the-art neurocritical care units (NCCU).
Despite this, no single brain monitoring method has yet emerged to supersede serial neurologic observations by a well-trained neuroscience critical care nurse. Many promising brain-monitoring technologies have been evaluated, but after initial enthusiastic use, have not continued to be used in patient care. Examples include jugular bulb oximetry and the “Neurotrend” system. Each of these represents ingenious technology able to generate meaningful data, yet they have failed to affect patient care in the long term. Nevertheless, technologies to monitor the brain continue to evolve and be developed, and much of what is done in the NCCU is to recognize changes in brain physiology and function and how the brain and the rest of the body interact. Ideally this is done through multimodality monitoring with a bioinformatics support platform, two concepts that will drive the future of monitoring in neurocritical care.
Aims of This Chapter
In an ideal system, information derived from monitoring should be specific, online or real-time, and filtered so that the pertinent information is presented, changes from the norm are identified, and measures to correct these abnormalities can be instituted. Ideally these methods should also include understanding the mechanisms associated with brain damage at the cellular level. This chapter briefly discusses the current neuromonitoring devices and techniques available, as well as new technologies projected to be available in the upcoming years. In addition it covers the role of industry in the advancement of new ideas and nanotechnology, and envisages the intensive care unit (ICU) of the future. Key issues relevant to modern ICUs are whether improved brain monitoring techniques can ultimately affect individuals’ quality of life and outcome.
The Basics: Glasgow Coma Scale and Physical Examination
The brain is 2% of total body mass yet receives 15% to 20% of the cardiac output. Because this tissue is extremely sensitive to hypoxia and ischemia, maneuvers to prevent critical reductions in arterial blood pressure (invasive arterial monitor or noninvasive cuff), peripheral arterial oxygen content (oxygen saturation monitor), intrinsic cardiac function (electrocardiogram), and pulmonary function (end-tidal CO 2 or arterial blood gas analysis) output assessment are important in critical care. A standardized neurologic exam (Glasgow Coma Scale [GCS]) introduced by Teasdale and Jennett in 1974 can be used for a basic assessment of brain function. It has prevailed as a simple yet critical bedside neuromonitoring technique because of its sensitivity to detect meaningful changes and its ability to be reproduced with a little variation.
When possible, new technologies should be compared to established gold standard techniques for specific measurement of changes in the injured brain. For example, a decrease in brain oxygen partial pressure reported by an intraparenchymal probe, should be confirmed (e.g., by xenon-enhanced computed tomography [Xe-CT] CBF) that those changes are actually accurate and relevant. With the development of portable computed tomography (CT) scanners that also allow evaluation of perfusion and flow, this may become a commonly accessible modality in many NCCUs.
Monitors of Brain Substrate Delivery
ICP Monitoring
Although ICP monitoring has been in use for greater than 50 years ( Fig. 48.1, A and B ), its utility is still questioned by some, who allege its use has not been demonstrated to save lives or improve outcome, in controlled trials. Such a trial sponsored by the National Institutes of Health (NIH) in Latin America to address this criticism was completed in late 2012. Nevertheless, these issues have been extensively reviewed in the latest revision of the severe TBI management guidelines, and level II evidence exists to supports ICP treatment in individuals with sustained ICPs greater than 20 mm Hg. With ICP and mean arterial blood pressure (MAP) measurements, cerebral perfusion pressure (CPP) can then be derived. The CPP value necessary to prevent ischemia varies according to the individual, but in general is between 50 and 70 mm Hg. Requirements for ICP monitors should be that the device is low cost, accurate, and reliable. Fluid-coupled external strain gauge intraventricular catheters traditionally cost less, but may experience significant measurement drifts over time and have a greater incidence of malfunction. Transducer-tipped devices (micro strain gauge [Codman, Raynham, MA] versus fiber-optic (Integra NeuroSciences, Plainsboro, NJ) cost slightly more, may be placed in either brain parenchyma or the ventricle, and may have greater accuracy over time (e.g., microstrain catheter), but cannot be recalibrated once placed. Wet/dry systems are now available, such as the Spiegelberg (Hamburg, Germany) transducer, which keep the compartments to measure ICP and drain CSF separate. These devices also have automatic zeroing functions. In conclusion, ICP monitoring currently plays a major role in monitoring brain status in the ICU. It should be considered in all patients where ICP is a question until alternative methods prove to be better. Developments will see an evolution of noninvasive devices, although at present none are able to replace invasive ICP monitors. In addition, it is well recognized that ICP is more than a number and that use of a single threshold at which to treat may be an oversimplification. Hence further evolution of ICP monitoring will include use of trend analysis and indices of autoregulation, pressure reactivity, and compliance to name a few.

SjvO 2 , P bt O 2 , CBF, NIRS
Because brain ischemia and hypoxia are common following TBI, the maintenance of tissue oxygenation above critical values is essential in modern NCCUs. This reduction in blood flow and oxygen delivery can occur within minutes after TBI; this stresses the importance of early intervention and initiation of monitoring. A mismatch or uncoupling between substrate demand and substrate supply also may be present because of the loss of cerebral autoregulation, and these changes can be present on a regional or more global scale. These tissues are thus at risk for permanent damage from critical reductions in the continuous delivery of glucose, oxygen, and other essential substrates. Aerobic metabolism becomes superseded by anaerobic mechanisms, ionic homeostasis is compromised, and calcium influx into cells along with potassium efflux causes widespread neuronal dysfunction and edema.
SjvO 2
Jugular venous oxygen saturation (SjvO 2 ) monitoring was implemented in the early 1990s as a promising method to detect post-traumatic changes in global cerebral oxygenation (see Chapter 32 ). The average SjvO 2 in normal subjects is 62%; a decrease less than 50% is defined as critical ischemia and is associated with a worse outcome. Mainstream use of this monitoring device has been limited, however, because of problems encountered with catheter migration or poor positioning, calibration errors (e.g., light absorbance), limited “time of good data quality,” and the inability to detect all causes of decreased brain oxygenation. Thus in some studies, more than 50% of measurements were erroneous, leading to far less use of the method in most centers. SjvO 2 also requires intensive supervision by specialized personnel. Future use of retrograde jugular catheters may also include the sampling of biomarkers, cytokines, or other factors such as endothelin that may influence CBF.
P bt O 2
A more direct approach to measure focal fluctuations in brain oxygen tension (P bt O 2 ) can be accomplished with Licox (GMS-Integra-Kiel, Mielkendorf, Germany) or Neurotrend (Codman, Raynham, MA) sensors (see Chapter 35 ). Optical sensors contained within the Neurotrend probe detect dye color changes resulting from differences in gas concentrations and pH of the surrounding tissues. One advantage of this particular device is the capability to measure oxygen concentrations and other modalities including carbon dioxide (P bt CO 2 ), pH, and temperature (T). The Licox sensor consists of a miniaturized Clark electrode with a polyethylene casing enclosing a solution of buffered potassium phosphates. Oxygen concentration is correlated with the electrical current created across a silver anode and gold-coated silver cathode. Sampling area is approximately 14 mm 2 , which is seven times the sampling area of the Neurotrend probe.
Critical thresholds of oxygen concentration have been determined for this technique. The oxygen value picked up by the sensor is a function of diffusion of oxygen from the erythrocyte or plasma to the extracellular space and it integrates all of the venous and arterial vessel oxygen tensions in the vicinity. Following placement, an increase of inspiratory oxygen fraction (FiO 2 ) to 100% can confirm the sensitivity of the device. With Neurotrend, sustained values of P bt O 2 that are less than 20 to 25 mm Hg are associated with poor outcome. Using Licox technology, P bt O 2 concentrations between 25 and 30 mm Hg are found in TBI patients with CPP and ICP in the normal range, but values less than 10 to 15 mm Hg are considered a sign of tissue at risk, and values less than 5 to 10 mm Hg indicate ongoing critical values, or, rarely, sensor malfunction. Furthermore, brain hypoxia (<10 mm Hg) appears to be an independent factor associated with poor outcome. Measures to increase P bt O 2 then need to be instituted. When PO 2 is maintained at normal values, it is associated with better outcomes in TBI patients. Persistent decreases in P bt O 2 also correlate with increases in brain lactate, and can result from with sustained ICP elevations, respiratory distress, and hypotension. In these studies, however, it was not possible to correlate brain PaCO 2 and pH with cerebral blood flow (CBF) and P bt O 2 . For example, van den Brink et al. in a study of 101 patients with severe nonpenetrating TBI (GCS ≤8), the magnitude and length of time of low P bt O 2 , measured by Licox were an independent factor associated with an unfavorable outcome and death. In addition, they observed that the devices demonstrated little zero drift and values were reliable over the time course of monitoring.
It remains unclear what is the optimal brain territory in which to place the probe. When placed in contused brain, increasing the FiO 2 does not result in increases in P bt O 2 to the same degree it does in noncontused brain, thus suggesting that CBF is too low to provide adequate substrate delivery in these contused areas. Therefore to recognize critical decreases of P bt O 2 some groups recommend placement in nonlesioned white matter in the frontal lobe for diffuse cerebral injury or on the affected side for a unilateral injury. Because of this heterogeneity of measured values between different areas of brain and the potential influence of local factors (e.g., microhemorrhages) that may contribute to the measurement, CT analysis of probe position is necessary. Most authors agree that P bt O 2 monitoring should not be used as a “stand-alone” monitoring technique and is best combined with ICP monitoring.
The Neurotrend sensors are no longer available because of commercial reasons. This emphasizes that although neuromonitoring technologies may be accurate and useful in clinical practice, small companies may be unable to sustain production costs for sophisticated microsensor systems without sufficient volumes of sales. The intrinsic conservatism of clinicians and hospital purchasing systems compound the problem. The award of Small Business Innovation Research (SBIR) grants may serve as a mechanism to help defray production costs, until uptake of new technologies achieves critical levels for commercial viability. In late 2012, an NIH-sponsored phase II randomized clinical trial to compare guided management of severe TBI using ICP alone to ICP and P bt O 2 (based on a Licox monitor) was halfway through patient recruitment. This trial hopefully will help clarify the utility and cost effectiveness of P bt O 2 monitoring.
Cerebral Blood Flow (LDF, TDF Probes)
Laser Doppler flowmetry (LDF) consists of an implanted subcortical fiber-optic laser probe (0.5-1 mm in diameter) that measures the shift of reflected laser light induced by movement of red blood cells (RBCs). Data are presented in real time, and variations in blood flow are monitored (see Chapter 31 ). The information is not quantitative, however, and represents only relative changes. Absolute values may be obtained when LDF is calibrated against other quantitative measures of CBF such as xenon-enhanced CT (see Chapter 27 ). Thermal dilution flow (TDF) methods (e.g., Bowman or Carter probes) are based on conductance of small amounts of heat between two small gold electrodes, one equipped with a sensitive thermocouple sensor (see Chapter 31 ). CBF is proportional to heat conductance, and the values are thus absolute, but the probes are usually configured for placement in deep white matter rather than gray matter. CBF changes therefore are less (e.g., normal CBF in white matter is ∼22 mL/100 g/min versus 80 mL/100 g/min in gray cortex). These CBF sensors usually are placed through a “bolt” system and can be placed at craniotomy (e.g., for aneurysm clipping). Clinical acceptance of both these monitors of CBF is limited in part because relative CBF values are reported, because of questionable reliability and validity, and because of questions on how to use the information. In particular it is difficult to make conclusions about CBF unless the state of metabolism is known. Nevertheless, use of these devices can supplement other monitors and provide information about autoregulation. Noninvasive methods to assess regional and global blood flow and oxygenation are under development and may one day replace current LDF and TDF monitors.
Noninvasive Monitoring of Brain Oximetry: NIRS
Near-infrared spectroscopy (NIRS) technology was initially pioneered by Jobsis and Chance, and is an emerging method to monitor cerebral oxygenation, blood flow, blood volume, cerebrovascular reactivity, and perhaps even edema in both adults and children (see Chapter 33 ). The basis of NIRS theory rests on the unique properties of light in the near infrared range to be scattered and absorbed when passing through biologic tissues by factors found within those tissues (e.g., chromophores such as water, lipids, cytochrome c oxidase, and various hemoglobin compounds). In basic terms brain NIRS involves transmission of light from an emitting source to a sensor placed nearby. Although transmission NIRS works well in neonates because of their semitransparent skull and scalp, NIRS monitoring in adults is degraded by the increase in skull and scalp tissue density. To overcome this limitation, reflectance mode NIRS is used, where an emitter and detector are separated by a specific distance on the scalp with the premise that a fixed amount of transmitted, reflected, and scattered light follows an elliptical path whose depth of penetration is proportional to the distance of separation between the emitter and the detector.
Devices Based on Absorption Measurements
The absorption spectra of deoxyhemoglobin (HHb) and oxyhemoglobin (HbO 2 ) in the infrared spectra between 700 and 900 nm has long been used to measure blood oxygen saturation. The attenuation of optical signals at convenient wavelengths in the near infrared range around the isosbestic point for hemoglobin (800 nm), are measured and used to determine the molar concentrations of HHb and HbO 2 and oxygen saturation in blood. This is possible because water and most other chromophores are functionally transparent or do not dramatically vary in absorption characteristics within the bandwidth interrogated ( Fig. 48.2 ).

The INVOS Cerebral Oximeter (Somanetics Inc., Troy, MI) uses a similar technique to estimate blood oxygen saturation in the brain. Optical transceiver pairs are placed on the left and right forehead over the prefrontal cortex. Typically, the attenuation of light signals at two wavelengths is used to estimate regional blood oxygen saturation. The separation between emitter-detector pairs is optimized to give as much signal as possible from tissues that underlie the skull.
These systems are used often during cardiac surgery to detect cerebral blood desaturation episodes and show promise in cerebral hematoma detection. However, there is limited enthusiasm for their use in NCCU monitoring, in part because of several limitations. First, attenuation due to scattering cannot be identified and subtracted. Second, signals from blood in tissues overlying the brain such as the scalp cannot be reliably identified and subtracted. Third, hair on the scalp also complicates measurements, and current systems only can be used in areas of the head not covered by hair such as the forehead. A few small clinical series, however, describe the potential application in both the operating room and in the ICU. For example, Brawanski et al. described a correlation between intraparenchymal oxygen probes and NIRS data in nine TBI patients. Kirkpatrick et al. found that NIRS registered changes in cerebral oxygenation that recovered once blood flow was restored during vessel cross-clamping in carotid endarterectomy. In 12 TBI patients the same investigators reported an increased frequency of correlative changes (e.g., decrease in peripheral saturation, intracranial hypertension, hyperemia) in NIRS versus SjvO 2 .
Several of the drawbacks of the first generation of cerebral oximeters have been addressed using techniques and equipment borrowed from radar and communications applications. This includes time of flight or time delay methods and multifrequency, multiwavelength phase modulation methods similar to the well-known time division multiplex (TDM) and wavelength division multiplex (WDM) methods used extensively in telecommunications applications. In short, a radiofrequency carrier wave is applied to the transmitted optical signal. Phase shifts in the returned optical signals coupled with the attenuations can be used to deconvolute scattering and absorption independently. In addition, the phase shift information can be used to spatially resolve the measurements and construct an image. In the future it is conceivable that this technique could be applied to the whole brain and provide a continuous image of brain oxygen saturation or a related parameter. However, no commercial product based on these techniques is available despite the significant body of research ( Fig. 48.3 ).

Devices Based on Light Scattering Measurements
The scattering of light in tissues is caused by refractive index mismatch between adjoining materials and structures within the tissue. In the near infrared bandwidth of 700 to 900 nm, scattering is the dominant effect thought to outstrip absorption by two orders of magnitude. In vivo scattering measurements show promise in the ability of NIRS to detect experimental brain edema in rodents. In these experiments the optical density of brain tissues was directly interrogated using NIRS signals and compared with the specific gravity of tissue samples harvested during the experiment and to ICP measurements. Water intoxication by direct parenchymal injection was used to induce brain edema and intracranial hypertension. The NIRS-measured optical density correlated with the specific gravity of the tissue and decreased with tissue swelling. In follow-up experiments, transgenic aquaporin-4 knockout mice and wild-type control animals were compared under the same conditions. The knockout mice had less decrease in optical density, suggesting that water uptake by cells may be responsible for the increased scattering and the subsequent decline in optical density of the tissue.
Further development of this device holds the promise of a continuous brain edema detector. If optical density is not affected by blood volume or indicates an increase in optical density, the technique may be able to differentiate between edema from cellular swelling or from vascular engorgement. The potential shortcomings associated with this technique include: (1) the effect of solutes such as mannitol and (2) that the measurement by its nature must be made in situ. Production of a “piggyback” device added to current intraparenchymal monitors may soon be feasible . In addition, prototype devices based on NIRS technology have been used for intracranial hematoma detection, and although at present these devices cannot replace CT they can in the future play an important role in the NCCU if the need for patient transport is avoided.
Microdialysis
Microdialysis (MD) was introduced to the clinical environment in the 1980s. It consists of a fine probe (0.62 mm in diameter) placed directly into the brain and may be combined with ICP and P bt O 2 probes. The probe is perfused with a sterile “mock extracellular fluid [ECF]” solution at a slow rate that allows continuous sampling of the parenchymal extracellular environment with the transfer of substances less than 20 kDa across a dialysis membrane. Assays are based on high-performance liquid chromatography (HPLC) techniques in small aliquots of dialysate fluid (60 µL). This allows identification and measurement of various molecules including glucose, lactate, potassium, pyruvate, nitric oxide, glutamate, and glycerol. The use of MD probes with much higher molecular weight cutoff (e.g., 70 or 100 kDa) allows protein fragments, and small peptides, such as cytokines (e.g., interleukin [IL]-1, IL-6, trophic factors, and amyloid precursor protein fragments) to be measured. Microdialysis has several limitations. First, measurements are representative of relative concentrations of the extracellular molecules and do not represent actual concentrations. This can limit data comparison between different machines and centers. Second, the information is not truly online, although newer devices, such as the CMA600 (CMA Microdialysis, Stockholm, Sweden) have shortened times of bedside substrate analysis to approximately 1 to 2 hours. Third, result specificity may be affected by cell edema, and changes in the ECF space around the catheter.
TBI, subarachnoid hemorrhage (SAH), and stroke among other disorders treated in the NCCU are associated with a cascade of events: excitatory amino acid (EAA) release, the opening of various ion channels that results in calcium influx and potassium efflux, failure of the sodium-potassium adenosine triphosphatase (Na + /K + -ATPase) transport, mitochondrial derangement, cytoskeletal breakdown, and a shift from aerobic to anaerobic metabolism that evolves over time (see Fig. 48.1 ). These changes have been evaluated in humans using microdialysis and show that adverse clinical events (e.g., elevated ICP, hypotension, or hypoxia) are associated with dialysate concentration increases (e.g., lactate, potassium, EAAs, or decreases such as glucose. There is a suggestion that the changes—elevated lactate-to-pyruvate (LP) ratios and glycerol—may precede an increase in ICP. In addition, a well-described and independent association exists between extracellular metabolic markers and outcome after TBI. However, it remains unclear whether treatment guided by microdialysis findings can alter outcome, although its use can be used to identify episodes of cerebral compromise not detected by conventional techniques (ICP and CPP) and to better stratify or target therapies based on metabolic disturbances.
Microdialysis in the NCCU is largely a research tool, and more research and technology development are required before it is adapted into routine clinical use. Development likely will include the ability to obtain both regional and nonregional information; the construction of analyzers (e.g., direct electrochemical detectors) that can give real-time readouts of an analyte such as lactate or glutamate; and evolution of dialysis membranes. However, the “best” analyte to be measured remains unclear.
Functional Monitoring: Glasgow Coma Scale, Electroencephalogram, Electrocorticogram, Evoked Potentials, Pupillometer
Glasgow Coma Scale and Neurologic Assessment
Outcome following acute brain injury including TBI, SAH, and stroke is associated with the initial neurologic condition. Hence assessment of consciousness, pupils, and limb movements is a foundation of neuromonitoring (see Chapter 10 ). This neurologic assessment is a continual process and can provide reliable and dynamic information about the injured brain. The examination, however, may be limited following intubation, sedation, and administration of chemical paralysis, and so standardized and objective scales are valuable. Since Teasdale and Jennett’s initial description of the GCS in 1974, it has evolved into the gold standard neuromonitoring technique because it is simple, reproducible, objective, and has limited intra- and interobserver variability. Other monitors therefore should be used with the GCS.
Brainstem Auditory Evoked Potentials
Brainstem auditory evoked potentials (BAEPs) assess the integrity of the auditory efferent pathways. Normally there are five waveforms: waves I through V represent signals generated at the vestibulocochlear nerve, cochlear nuclei, superior olivary complex, lateral lemniscus, and inferior colliculi, respectively. BAEPs are useful in posterior fossa surgery but have a limited role in assessment of patients in the NCCU, although they may help confirm the diagnosis of brain death (e.g., absence of all waveforms or presence of wave I). On the other hand, somatosensory evoked potentials (SSEPs) may play a role in prediction of outcome. This is further reviewed in Chapter 24 , and guidelines on the use of clinical neurophysiology in the ICU are available.
Electroencephalogram, Electrocorticogram, Cerebral Function Monitoring: Seizures and Spreading Depression
Subclincal, nonmotor, or partial seizures may occur in about 20% of patients in the NCCU if looked for, and these nonconvulsive seizures can adversely influence outcome. Overt motor seizures, however, may be masked by chemical paralysis, so monitoring for epileptiform activity can be an important adjunct to ICP, brain oximetry, or CBF monitors. Electroencephalogram (EEG) data also are associated with outcome and can be used to guide therapeutic interventions before irreversible damage ensues. The presence of epileptiform activity also is linked to episodic increases in ICP and the LP ratios. Finally, EEG data can be used as a surrogate endpoint to test the efficacy of new drugs without having to wait for functional outcome measures (typically 6 months to 1 year).
EEG monitoring is noninvasive, and machines used in the NCCU today are small and portable, contain data reduction software (e.g., microprocessor technology that splits information into spectra dependent on frequency, amplitude, and location), provide continuous data, and eliminate the need for a full-time technical assistant at the bedside. The simplest form of EEG monitoring includes a noninvasive strip of 2 to 4 electrodes (e.g., bispectral index [BIS]) that analyzes the depth of sedation and whether seizure activity is present. These findings correlate with the GCS. However, BIS cannot regionalize information. Larger EEG units including 8- to 14-channel 10- to 12-electrode montages provide better spatial resolution and can collect specific information such as alpha, delta, beta, and theta proportions of the raw EEG. Sophisticated units display complex information in more simple formats that are easy to read and interpret in the NCCU and can present the information in color spectra similar to the appearance of a positron emission tomography (PET) scan.
Following craniotomy, invasive EEG monitoring is feasible with placement of an electrocorticogram (ECoG) recording strip or grid on the cortex near the injury site. The use of a single linear strip facilitates removal at the bedside once the monitoring period is complete. Collected data can be stored and analyzed offsite by specialized personnel. Use of ECoG provides insight into cortical spreading depression (CSD) and peri-infarct depolarization (PID). CSD consists of a wave of depressed cortical activity or amplitude that spreads slowly across cerebral gray matter. The depression is accompanied by a transient loss of ion homeostasis, changes in glucose and lactate, and an increase in CBF and oxygen followed by oligemia. ECoG changes are similar to those recorded during PIDs, which occur in the periphery of infarct regions. However, PID and CSD are different, in that PID may contribute to irreversible ischemic damage. Whether CSD harbors long-term effects in patients and what they are is still to be fully elucidated.
Animal studies dating back 60 years show that several mechanisms including potassium and glutamate in the ECF can influence CSD. However, the presence of CSD in humans after TBI has only recently been confirmed and depends in part on the ability to place ECoG monitors in perilesional zones rather than standard frontal sites that are often distant from a lesion. CSD may be observed in a third of these patients and is more likely to occur in younger patients. In addition, CSD may occur with or precede a seizure, although this observation is inconsistent and further study of this relationship is required. Both CSD and PID events can be attenuated with N-methyl-D-aspartic acid receptor blockade in animals, and so monitoring for these changes in humans may provide a selection tool for those patients who could benefit from these therapies in the future. Implantable fiber-based optical probes also have been used to detect preictal changes in the rodent hippocampus and determine whether scattering coefficients can predict electrographic seizure onset in vivo.
Pupillometer
Pupillary assessment is important when defining prognosis after brain injury and to identify herniation; this component of the neurologic assessment has the potential to become “automated” and more quantitative and sensitive through use of a pupillometer. The neurologic pupillary index (NPi) is an objective algorithm based on the pupillary indices obtained from healthy subjects that uses a handheld digital camera pupillometer that automatically tracks and analyzes pupil dynamics (NeurOptics Inc., Irvine, CA) ( Fig. 48.4 ). This technology has the potential to limit subjective descriptions of pupillary change and reduce interobserver variability. The results of pupil assessment are stored in the device’s memory and projected onto a liquid crystal display (LCD) screen. Use of the pupillometer may provide an early warning of ICP changes that cannot be appreciated by visual inspection by the health care provider alone (i.e., clinical measures could be instituted early, to prevent ICP peaks and CPP reduction).

New Technologies That May Change Neuromonitoring: Biomarkers, Nanotechnology, Microelectronics, Wireless Systems, “Lab on a Chip”
Biomarkers: The Search for a Troponin Equivalent in the Brain
The term biomarker has different meanings to different users, but in the NCCU it implies a substance or parameter that represents either organ outcome or function. Ideally biomarkers also need to respond to treatment. Two examples are the reduction in viral load levels and increase in CD4 cells in human immunodeficiency virus (HIV) with proper antiretroviral treatment and the reduction in cardiac specific troponin levels following reestablishment of blood flow to the heart. The ideal “brain biomarker” is still being sought (see Chapter 18 ) but has the potential to improve understanding of the mechanisms associated with cellular, organ, and systemic damage to facilitate future drug development and to alert clinicians when changes occur to guide management. Similar to how cardiac troponins are specific to cardiac muscle injury, the ideal neurologic biomarker should be accessible, specific to the nervous system, identify progression of secondary damage, and predict outcome. Ideally it should appear in blood and CSF immediately after an injury. Serum biomarkers are advantageous because of sampling ease.
Potential biomarkers include cytokines (e.g., IL-6, IL-1, tumor necrosis factor [TNF], and transforming growth factor-β [TGF-β]) that increase in blood 48 hours post injury, brain-specific creatine kinase (CK), glial fibrillary acidic protein (GFAP), lactate dehydrogenase (LDH), myelin basic protein (MBP), neuron-specific enolase (NSE), S-100β (protein expressed constitutively by brain astrocytes), and spectrin breakdown products (SBP 120), among others, or a combination of these markers. NSE, a glycolytic enzyme, appears rapidly in serum after brain injury and is specific to brain tissue but results are conflicting and in some studies has failed to separate persons with mild TBI from normal controls. S-100β also has undergone extensive study and its levels are associated with the GCS, imaging, and outcome. However, it also is found in tissue outside the brain, adipose tissue, and chondrocytes. GFAP is released following TBI but not after multiple trauma without TBI; the GFAP levels correspond to TBI severity and outcome. Alpha II-spectrin is a cytoskeletal protein found in axons and presynaptic membranes that is cleaved by caspase-3 and calpain, two enzymes that are activated following TBI and can be measured in both blood and CSF. This protein is elevated in patients following TBI compared with controls, and secondary increases in CSF are observed with clinical deterioration.
Despite much research there is no ideal biomarker for practical clinical use in TBI. It also is conceivable that rather than a single biomarker, a panel of several biomarkers may be important. Proteomic analysis may provide a vehicle for new molecular discovery. A large multicenter trial is underway to examine multiple biomarkers in TBI (Banyan Biomarkers, Inc. Alachua, FL). Once an ideal biomarker is defined, an enzyme-linked immunosorbent assay (ELISA) or electrochemical detection system could be developed, and a “chip” or kit devised to allow cheap, rapid, serial measurements at the bedside. The U.S. military has invested heavily in research designed to produce such a biomarker detection device that is the size of a cell phone and can be deployed with medics in combat zones.
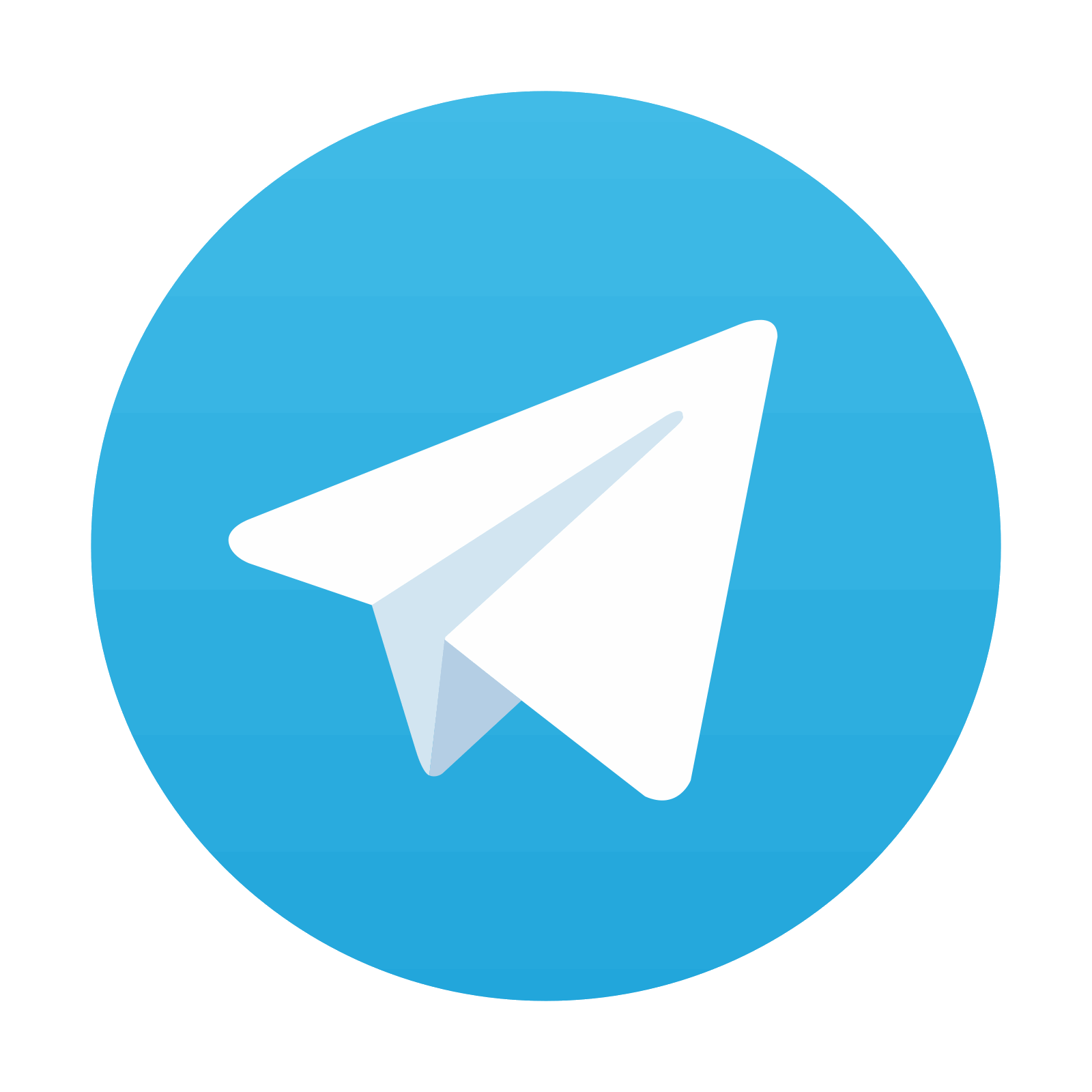
Stay updated, free articles. Join our Telegram channel
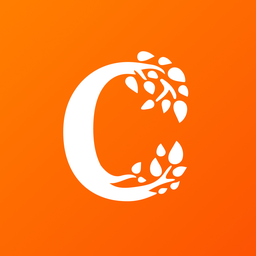
Full access? Get Clinical Tree
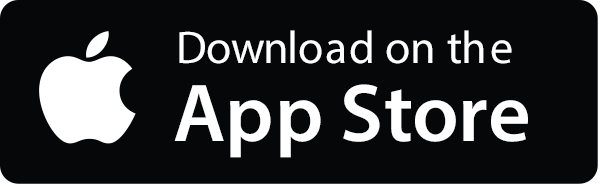
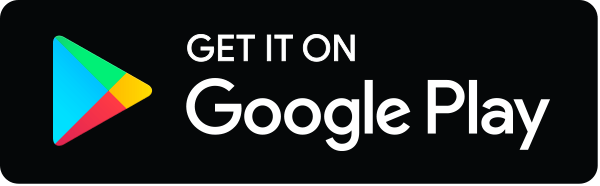