Fig. 3.1
Morphological metabolic coupling between the presynaptic terminal and the astrocytic process, i.e., glial microdomain (Grosche et al. 1999), but with a new metabolic course. These two compartments are considered to have equal volumes. Metabolite molecules M are transported into the astrocytic process (some may also be produced locally) through a conduit depicted by the narrow passage (J 2). The flux in the pathway to the presynaptic terminal (J 1) is mediated by transporters, depicted by spheroid symbols that permeate metabolite molecules M
One mechanism seems to involve G-protein coupled receptor (GPCR)-mediated processes. It has been known for some time that astroglial β-adrenergic receptors (β-ARs) functionally regulate astrocyte cellular morphology (Hatton et al. 1991). An increase in intracellular adenosine 3ʹ,5ʹ cyclic monophosphate (cAMP) production on β-AR stimulation induces astrocyte stellation (Fig. 3.2), i.e., transformation from a flattened polygonal morphology to a stellate process-bearing morphology (Shain et al. 1987; Bicknell et al. 1989). How vesicle traffic contributes to these processes is largely unclear. β-ARs are abundant on astrocytes in both white and grey matter of the brain (Aoki 1992; Sutin and Shao 1992; Zeinstra et al. 2000; Catus et al. 2011) and are likely involved in a number of pathologic conditions. It is likely that during the early stages of AD, when a reduction of noradrenaline was reported (Hammerschmidt et al. 2013), this may result in reduced cAMP signaling and in astrocyte atrophy, the latter as observed in the triple transgenic animal model of AD (Olabarria et al. 2010). Such astrocytic atrophy may lead secondarily to synaptic loss due to insufficient metabolic support of synapses by astrocytes. In this particular case, astrocytes signal to neighboring cells likely using gliotransmitter vesicles.
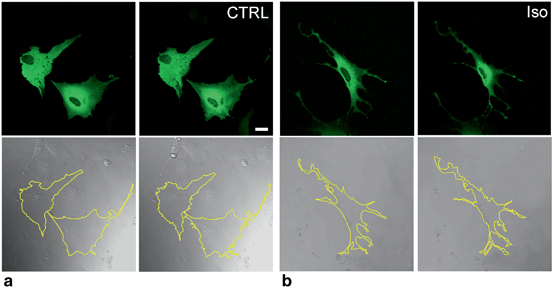
Fig. 3.2
Morphological changes in astrocytes (stellation) induced by β-AR activation, which increases cAMP. a, b Green fluorescing astrocytes transfected with the cAMP nanosensor Epac1-camps (Nikolaev et al. 2004) (top) and their corresponding differential interference contrast images (bottom) before (left) and within 30 min after (right) the addition of (a) extracellular solution as control (CTRL) and (b) 1 µM β-AR agonist isoprenaline (Iso), which increases intracellular cAMP levels through activation of β-ARs in astrocytes. b Rapid change in the cross-sectional area and perimeter (see the yellow outline in the bottom panels) of astrocytes, where thinning of processes indicates astrocyte stellation. The perimeter of individual cells expressing Epac1-camps was traced using LSM510 META software, which also outlines the cross-sectional area of the cell. Scale bar: 20 µm
3.3 Gliotransmitter Vesicles
Gliotransmitters are chemicals released from glial cells and are synthesized by and/or stored in glia (Parpura and Zorec 2010) . Storage of gliotransmitters in membrane-bound vesicles as opposed to having them stored in the cytoplasm provides several advantages for cell-to-cell communication (Guček et al. 2012). In astrocytes , several types of gliotransmitter vesicles have been reported containing the amino acids glutamate and d-serine (Parpura et al. 1994; Bezzi et al. 2004; Kreft et al. 2004; Montana et al. 2004, 2006; Martineau et al. 2008, 2013), ATP (Coco et al. 2003; Pangrsic et al. 2007) , and peptides such as ANP (Krzan et al. 2003; Kreft et al. 2004), BDNF (Bergami et al. 2008), and tissue plasminogen activator (tPA) (Cassé et al. 2012). Several vesicle mobility experiments have been performed on primary cultured astrocytes to explore how vesicle mobility is altered under different physiologic and pathologic conditions.
It is not even a decade since the first spontaneous mobility of membrane-bound vesicles in astrocytes was described (Potokar et al. 2005), and subsequently confirmed (Crippa et al. 2006). To determine vesicle mobility, several parameters such as the total track length, the path a vesicle travels in a given period of time, the average velocity, the displacement, and the directionality index (ratio between the maximal displacement/total track length) can be measured. Maximal displacement represents a measure of the maximal net translocation of vesicles (Wacker et al. 1997). Consistent with other cell types (Burke et al. 1997; Tvaruskó et al. 1999; Duncan et al. 2003; Potokar et al. 2005), two distinct modes, directional and nondirectional, of vesicle mobility have been described in astrocytes, and these modes of mobility were able to switch while a vesicle was being observed (Potokar et al. 2005) (Fig. 3.3a) .
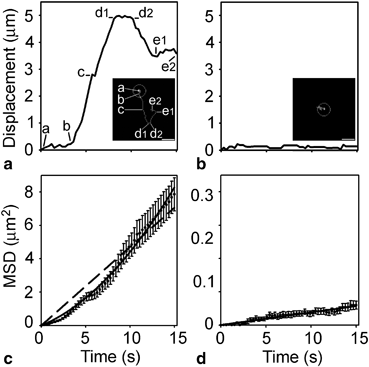
Fig. 3.3
Single-vesicle mobility tracks revealing directional and nondirectional vesicle mobility. a Displacement from the origin vs. time for a directional vesicle. The first quarter of the vesicle’s mobility tracking time from the origin consists of almost constant displacements (a to b) and, during this time, the vesicle remains close to the origin of tracking (see inset (a)). In the next third of the tracking time (b–d1), vesicle displacements increase rapidly in a preferential direction with a brief pause (c). After a short period with equal displacements (d1–d2), the vesicle seems to move backwards, however, apparently not on the same track (d2–e1 and inset). b Displacement from the origin for a nondirectional vesicle. Minor mobility was observed and the vesicle did not translocate far from the origin of tracking (see inset). The mean square displacement (MSD) shown in (c, d) was calculated according to the equation MSD = [d(t) – d(t + ∆t)]2. (c) The MSD of directional vesicles. The dashed line represents a linear function fitted to the data using an equation with the form MSD (μm2) = (0.4702 ± 0.0099) × time (s). The upwardly curving line represents a quadratic function fitted to the data following the equation MSD (μm2) (0.2189 ± 0.0148) × time (s) + (0.0221 ± 0.0013) × time2 (s2). d The MSD of nondirectional vesicles. The linear function was fitted to the data following the equation MSD (μm2) = (0.0038 ± 0.0001) × time (s). Bar: 2.5 μm. (Reproduced from Potokar et al. (2005); with permission)
3.3.1 Amino Acid-loaded Vesicles
In astrocytes , glutamate is packaged into vesicles by the vesicular glutamate transporters (VGLUTs) VGLUT1, VGLUT2, and VGLUT3 (Danbolt 2001; Parpura and Zorec 2010) . Although the existence of VGLUT1 in mouse astrocytes has been questioned (Li et al. 2013), VGLUT1-containing vesicles in rat astrocytes are small and electron lucent, with an estimated diameter of ~ 30 nm in situ (Bezzi et al. 2004) and ~ 50 nm when they recycle (Stenovec et al. 2007), but larger sizes have also been reported (Chen et al. 2005; Malarkey and Parpura 2011). Why different vesicle diameters have been reported for glutamatergic vesicles is not known but this may also be associated with different microscopy techniques used by different investigators. Figure 3.4 shows an example of vesicles fluorescently labeled by d-serine antibodies in fixed cultured astrocytes examined by confocal and stimulation emission depletion microscopy (STED, a superresolution fluorescence microscopy technique (Hell and Wichmann 1994; Jorgacevski et al. 2011)) that presents new possibilities to resolve the question of the different diameters reported for gliotransmitter vesicles in astrocytes.
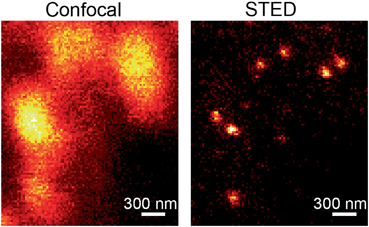
Fig. 3.4
Comparison of vesicles loaded with d-serine recorded by confocal microscopy (left) and by STED (right). Note that vesicles, which are positioned at a distance less than about half the emitting light wavelength (~ 300 nm), cannot be resolved with diffraction limited confocal microscopy. On the other hand, STED microscopy, which is the first far field microscopy to break the diffraction limit, successfully resolves individual vesicles
In addition to studies of spontaneous vesicle mobility, experiments on astrocytes have revealed that vesicle mobility may be altered in stimulated cells. This was observed when glutamatergic vesicles were labeled in vivo using a novel approach (Stenovec et al. 2007) based on the fact that after Ca2+-dependent exocytosis (Parpura et al. 1995; Jeftinija et al. 1996; Zhang et al. 2004a, b), exocytotic vesicles are endocytosed (Stenovec et al. 2007). Before entering the endocytotic pathway, exocytotic vesicles may enter several rounds of recycling, whereby the transient exocytotic fusion pore reopens several times. Vesicles that transiently expose their lumen to the extracellular space may take up fluorescently labeled antibodies against VGLUT1. These antibodies were raised against the amino acid residues that were thought to be present only in the cytoplasmic part of VGLUT1 transporter protein, but these are likely also present in the vesicle lumen in native vesicles, because anti-VGLUT1 antibodies label the luminal part of vesicles (Almqvist et al. 2007; Stenovec et al. 2007). At higher intracellular concentrations of Ca2+ ([Ca2+]i) induced by4 µM ionomycin or 1 mM ATP, immunolabeling was more pronounced and the directional mobility of VGLUT1 vesicles was increased. Together with directionality, the fraction of fast-moving vesicles (> 0.05 μm/s) increased at higher [Ca2+]i. These effects were absent in the cells preloaded with high-affinity Ca2+ buffer (BAPTA-AM). Microtubules, actin, and vimentin filaments likely play a role in the mobility process of VGLUT1 vesicles, because the disruption of actin attenuated their mobility (Stenovec et al. 2007). As discussed by Stenovec et al. (2007), regulation of vesicle mobility after vesicle retrieval may be involved in various aspects of physiology, such as synaptic plasticity (Aravanis et al. 2003), silent synapses (Gasparini et al. 2000), astrocyte-to-neuron communication (Haydon 2001; Volterra and Meldolesi 2005), and possibly more widely in cell biology in the genesis and removal of vesicles from the plasma membrane (Pelkmans and Zerial 2005). The stimulation-enhanced mobility of glutamatergic vesicles contrasts the stimulation-induced attenuation of mobility of peptidergic vesicles and endosomal structures, which likely plays an important role under pathologic conditions (Potokar et al. 2008, 2010, 2011).
3.3.2 ATP-loaded Vesicles
ATP, an essential component of long-range Ca2+ signaling in the nervous system (Zimmermann 1994), is also an important astrocytic gliotransmitter (Parpura and Zorec 2010) and one of the major extracellular messengers for interastrocyte Ca2+-mediated communication (Guthrie et al. 1999; Wang et al. 2000) . In addition to nonvesicular modes of ATP release, such as the release of ATP from astrocytes mediated by the connexin hemichannel (Stout et al. 2002; Stehberg et al. 2012), volume-sensitive organic osmolyte and anion channels (Blum et al. 2010), Ca2+-dependent exocytotic ATP release from astrocytes has also been confirmed (Parpura and Zorec 2010). ATP-loaded astrocytic vesicles seem to be heterogeneous. So far in astrocytes, the vesicular distribution of ATP has been shown to overlap with the marker of dense-core granules in the hippocampus, secretogranin II (Calegari et al. 1999; Coco et al. 2003) and in lysosomes (Jaiswal et al. 2007; Zhang et al., 2007; Li et al. 2008; Verderio et al. 2012). ATP seems to be co-stored together with classic neurotransmitters (acetylcholine in neurons and noradrenaline in neurons and chromaffin cells) (Zimmermann 1994) or with peptides (Calegari et al. 1999; Belai and Burnstock 2000; Bodin and Burnstock 2001; Coco et al. 2003; Pangrsic et al. 2007). In neonatal cortical rat astrocytes, ATP-containing vesicles seem to substantially co-store ANP (39 ± 7 %) (Pangrsic et al. 2007). Under spontaneous conditions, most of the ATP vesicles were located in close proximity to the plasma membrane (up to 150 nm) and this coincided with the observation that quinacrine-loaded vesicles displayed mainly nondirectional spontaneous mobility and only 4 % of vesicles were highly mobile (directional mobility). High [Ca2+]i affected both types of vesicle mobility and completely abolished directional mobility. After a triggered increase in [Ca2+]i, less ATP vesicles were observed in the cells, likely due to Ca2+-activated discharge of the fluorescent/quinacrine cargo by regulated exocytosis. This effect was obstructed by the presence of the dominant-negative soluble N-ethylmaleimide-sensitive factor (NSF) attachment protein receptor (SNARE) domain peptide, which interferes with the formation of the SNARE complex (Zhang et al. 2004b; Pangrsic et al. 2007).
ATP is considered to be a major gliotransmitter in the propagation of calcium waves among astrocytes (Haydon 2001), and in the modulation of neuronal activity (Zhang et al. 2003; Pascual et al. 2005; Haydon and Carmignoto 2006) , the exocytotic release of ATP may play a role in the delivery of this gliotransmitter to the extracellular milieu as a signaling messenger for intercellular communication. In the dominant-negative SNARE mouse model, it was shown that ATP release is reduced and this prevents tissue damage after a stroke (Hines and Haydon 2013).
3.3.3 Peptide-loaded Vesicles
ANP, which is stored in membrane-bound vesicles, was first shown to be released by Ca2+-dependent exocytosis from astrocytes (Krzan et al. 2003). The cytoplasmic mobility in the secretory pathway was monitored using ANP.emd fluorescent recombinant protein (Han et al. 1999), whereas the mobility in the recycling pathway was monitored using an immunolabeling approach in living cells (Potokar et al. 2008).
The major difference between the mobility of ANP vesicles monitored in the different pathways in rat astrocytes was the traveling speed. In vesicles traveling from the cytoplasm to the plasma membrane, the speed was 0.4 ± 0.007 µm/s (nondirectional vesicles 0.3 ± 0.005 µm/s, directional vesicles 0.5 ± 0.01 µm/s), indicating that all vesicles were mobile but some displayed directional motion. Such rapid vesicle mobility is comparable with the directional movement of vesicles in some neurons (Potokar et al. 2005). However, their mobility was significantly attenuated after depolymerization of microtubules, actin filaments, and intermediate filaments (IFs) to 0.30 ± 0.0003 µm/s, 0.08 ± 0.01 µm/s and 0.21 ± 0.01 µm/s, respectively (Potokar et al. 2007). In mouse astrocytes, the measured parameters of mobility were lower compared with rat astrocytes (Potokar et al. 2013b). The slight difference in speed was also recorded between wild-type (WT) astrocytes and astrocytes without IFs, and the extent of directional mobility was also lower in astrocytes without IFs (Potokar et al. 2007). These data support the hypothesis that IFs are required for long-range directional vesicle mobility by acting as three-dimensional conduits. The importance of astrocytic IFs in vesicle mobility under pathologic conditions has also been confirmed by more recent studies (Vardjan et al. 2012).
The mobility of ANP vesicles in the recycling pathway has been monitored in rat astrocytes. Vesicle recycling has been considered for secretory granules, which are released by stimulated exocytosis (Taraska et al. 2003). During this process, the granule remains intact, except for the loss of the contents and some of the membrane proteins. Recycling occurs when the fusion pore is rapidly resealed in the exocytotic process and the vesicle is retrieved into the cytoplasm without intermixing of membranes and without collapse of the vesicle membrane into the surface membrane (Jahn and Südhof 1999; Valtorta et al. 2001; Taraska et al. 2003). When studying recycling ANP vesicles, they exhibited one order of magnitude slower mobility than secretory ANP vesicles (Potokar et al. 2013b). What is the physiologic significance of these results? The mobility of vesicles retrieved from the plasma membrane after exocytotic fusion is likely related to the efficiency of vesicle cargo discharge. If sufficient time is allowed (during attenuated vesicle mobility), the vesicle cargo can be completely discharged from the vesicle lumen, especially if peptides in the vesicle are aggregated into dense matrices (detected as electron-dense material on electron microscopy) and their discharge is inefficient unless the vesicles exhibit a fusion pore that permits prolonged discharge of the vesicle cargo. Furthermore, brain ANP content is significantly increased after experimental brain infarction, but not after brain hemorrhage, after contusion and in controls, indicating that ANP-positive astrocytes may increase in number and may be involved in the regulation of the cerebral blood flow in the infarcted brain area (Nogami et al. 2001). The altered cerebral blood flow thus also underlies enhanced delivery of ANP vesicles to the plasma membrane and/or release of ANP from astrocytic vesicles.
In addition, a specialized form of bidirectional communication involving signaling peptides exists between neurons and astroglia . BDNF secreted from neurons in its precursor form (pro-BDNF) is cleared from the extracellular space into nearby astrocytes, which internalize it via formation of a complex with the pan-neurotrophin receptor p75 and subsequent clathrin-dependent endocytosis. Endocytosed pro-BDNF is then routed into a fast recycling pathway for subsequent SNARE-dependent secretion triggered by glutamate (Bergami et al. 2008). Similarly, tPA appears as an element of the crosstalk between neurons and astrocytes. tPA released by neurons is constitutively endocytosed by astrocytes via the low-density lipoprotein-related protein receptor, and is then exocytosed in a regulated manner. Here, however, the exocytotic recycling of tPA by astrocytes is inhibited by extracellular glutamate. Kainate receptors of astrocytes act as sensors of extracellular glutamate and, via a signaling pathway involving protein kinase C, modulate the exocytosis of tPA (Cassé et al. 2012). Apart from this, BDNF is the most prevalent growth factor in the CNS and is widely implicated in psychiatric diseases , such as major depressive disorder (MDD), schizophrenia, addiction, and Rett syndrome (Autry et al. 2011). Notably, N-methyl-d-aspartate receptor (NMDAR) antagonists may produce fast-acting behavioral antidepressant effects in patients with depression and studies in mouse models indicate that these effects depend on the rapid synthesis of BDNF. At the cellular level, the blockade of NMDAR deactivates eukaryotic elongation factor 2 (eEF2) kinase/CaMKIII, resulting in reduced eEF2 phosphorylation and de-suppression of translation of BDNF (Autry et al. 2011). Although the regulation of protein synthesis has been identified as a valuable therapeutic target in the treatment of MDD, one cannot rule out the possibility that fast-acting antidepressants affect trafficking and/or release of presynthesized BDNF from brain cells; patients with depression report alleviation of MDD symptoms within 2 h of a single, low-dose, intravenous infusion of the antidepressant drug (Zarate et al. 2006).
Whether vesicles in intact tissue exhibit similar mobility to cultured cells was tested by labeling recycling vesicles in astrocytes in hippocampal tissue slices, because brain tissue slices represent a preparation that is physiologically closer to that occurring in vivo, i.e. cell-to-cell contacts and tissue architecture are preserved as present in the brain (Potokar et al. 2009). We incubated brain slices from mice with antibodies either against ANP or against VGLUT1. Vesicles in astrocytes from the CA1 region of the hippocampus were recorded. The recording of vesicle mobility was performed with two-photon microscopy. The fluorescent puncta exhibited two types of mobility: nondirectional and directional. The average velocity of ANP-containing granules in slices from mice was approximately 0.04 µm/s, which is similar to that reported for recycling ANP granules in rat primary astrocyte cultures (0.06 µm/s) (Potokar et al. 2008), but one order of magnitude slower than the velocity of pro-ANP.emd-labeled granules (Potokar et al. 2005, 2007), where the average velocity was 0.4 µm/s. The mobility of VGLUT1 vesicles was analyzed similarly. The VGLUT1 vesicles in the slices were slightly slower than the ANP vesicles; their average velocity was approximately 0.03 µm/s. The velocity of recycling VGLUT1 vesicles in the slices was also slightly slower than recycling VGLUT1 vesicles from rat primary astrocyte cultures (0.05 µm/s) (Stenovec et al. 2007). Although vesicle mobility may differ in different brain regions, these results show that the experimental data obtained from cultured astrocytes closely resemble the properties of vesicle mobility observed in tissue slices.
3.4 Other Vesicles
The mobility properties of endosomes and lysosomes have been described in detail in mouse (Potokar et al. 2010) and rat astrocytes (Stenovec et al. 2011). These vesicles were labeled by LysoTracker dye (Ly) and exhibited slow mobility compared with other vesicle types (Potokar et al. 2013b). The direction and speed of Ly vesicles was shown to be influenced by the absence of astrocytic IFs. The trafficking of Ly-labeled vesicles seems to be regulated differently from glutamate-containing (VGLUT1-positive) and peptide-containing (ANP-positive) vesicles under different physiologic conditions. Cell stimulation to trigger an increase in [Ca2+]i significantly reduced the mobility of Ly-labeled vesicles in WT astrocytes but not in astrocytes devoid of IFs (GFAP − / − Vim − / − astrocytes) (Pekny et al. 1998; Eliasson et al. 1999; Wilhelmsson et al. 2004) . Moreover, stimulation-dependent regulation of VGLUT1- and ANP-positive vesicles was attenuated by the absence of IFs. Because these filaments get overexpressed under pathologic conditions (Eliasson et al. 1999), it is likely that the traffic of distinct vesicle types is altered under these conditions (Potokar et al. 2010), likely leading to vesicle traffic jams (Potokar et al. 2011).
The regulation of endosome and lysosome mobility may exhibit completely different properties in pathophysiologic states. For example, if purified IgG antibodies harvested from patients with sporadic amyotrophic lateral sclerosis (ALS) are applied to astrocytes, the mobility of Ly-labeled compartment(s) is transiently increased, likely in a Ca2+-dependent manner, indicating that acidic compartments may not represent a functionally homogeneous subcellular compartment, although endosomes and lysosomes were stained predominantly (Stenovec et al. 2011). The altered mobility is likely associated with altered Ca2+ homeostasis by ALS IgGs (Milošević et al. 2013). How do these results relate to the disease? ALS is a complex, incurable, and non-cell autonomous degenerative disease that affects upper and lower motor neurons located in a neighborhood enriched with nonneuronal cells; it occurs in adulthood (Haidet-Phillips et al. 2011) with a projected lifetime risk of 1 in 2000 (Eisen 2009). The hallmark of ALS is selective death of motor neurons, although glial cells are also affected. In ALS, astrocytic function is compromised in several ways that impair neuronal survival and includes deficient release of (i) neurotrophic factors (Ekestern 2004); (ii) release of nerve growth factor or extracellular mutant superoxide dismutase 1 (SOD1) (Pehar et al. 2004; Urushitani et al. 2006); (iii) insufficient clearance of glutamate from the synaptic cleft, due to reduced density and loss of EAAT2 (Rothstein et al. 1995). Disturbance of the physiologic balance between neurons and astrocytes may therefore play a key role in motor neuron degeneration in ALS (Van Damme et al. 2005). In addition, activation of a systemic immune response in patients with ALS (Zhang et al. 2005) may play a role in the continuing pathology of ALS, once the BBB is compromised (Garbuzova-Davis et al. 2007). Correspondingly, motor neurons survived less when cocultured on astrocytes expressing the mutant form of Cu-Zn SOD1, as in the familial type of ALS, than on WT astrocytes (Di Giorgio et al. 2007). The application of conditioned medium from mutant SOD1-expressing astrocytes decreased the survival of motor neurons, suggesting the presence of astrocyte-secreting molecules that kill neurons (Nagai et al. 2007). Alterations in vesicle dynamics may thus reflect changes associated with the progression of the disease and may offer possibilities for the development of new diagnostic tests.
3.4.1 Aquaporin Transporting Vesicles
Recently, it was shown that the water channel AQP4 is also trafficking in vesicles that exhibit the properties of endosomes and lysosomes (Potokar et al. 2013a). This key molecule is involved in brain water homeostasis and is one of the three AQPs identified in brain cells in vitro and in vivo (Hasegawa et al. 1994; Jung et al. 1994; Nielsen et al. 1997; Badaut et al. 2002; Amiry-Moghaddam and Ottersen 2003). AQP4 isoforms in rodent and nonhuman primate brains are the most strongly expressed in astrocytic end feet surrounding the BBB (Nielsen et al. 1997; Nagelhus et al. 1998; Neely et al. 1999; Arciénega et al. 2010) and have also been identified in astrocytic processes in contact with synapses (Nielsen et al. 1997; Badaut et al. 2000a, b). Several studies have suggested an important role of AQP4 in water transport in several processes including astrocyte swelling and brain edema formation/resolution under various pathologic conditions, both in vitro (Yamamoto et al. 2001; Arima et al. 2003) and in vivo (Manley et al. 2000; Ke et al. 2001; Papadopoulos et al. 2004). Water transport through the cell membrane may be regulated by the permeability properties of AQP4 (Gunnarson et al. 2008; Nicchia et al. 2011), the heterogeneity of AQP4 crystalline-like orthogonal arrays of particles (Hirt et al. 2011), and, as recently suggested, by the mobility of the AQP4 vesicles that are delivered to and from the plasma membrane (Potokar et al. 2013a). The properties of AQP4 vesicle mobility are described in a study by Potokar et al. (2013a). AQP4 is one of the newly described basic AQP4 isoforms (Moe et al. 2008). In unstimulated conditions, the mobility of AQP4 vesicles resembled the mobility of slow recycling and endosomal vesicles (Stenovec et al. 2007; Potokar et al. 2008, 2010). After dibutyryl-cAMP treatment, a model to induce reactive astrocytosis, an increased AQP4 signal was measured at the plasma membrane after 15 min (and remained increased even after 24 h) and the mobility of AQP4 vesicles was affected (Potokar et al. 2013a). These data indicate that the regulation of vesicle mobility in the relatively short time scale is an important regulatory mechanism to alter the delivery and removal ratio of AQP4 vesicles at the plasma membrane in reactive astrocytes. Decreased mobility with significantly lower directionality might contribute to restraining the AQP4 vesicles near the plasma membrane and may also be linked to dibutyryl-cAMP-induced rearrangements of the F-actin cytoskeleton mesh already considered to be one of the major factors responsible for increased AQP4 plasma membrane localization (Nicchia et al. 2008).
During the early stages of brain edema formation, astrocytes swell (Papadopoulos et al. 2004; Nase et al. 2008; Risher et al. 2009). A reduction in osmolarity triggers an increase in soma volume; this has been measured in tissue and in cultured rat astrocytes (Takano et al. 2005; Pangrsic et al. 2006; Thrane et al. 2011). The increase in cell volume may be accompanied by an increased rate of insertion of exocytotic vesicles in the membrane (Pasantes-Morales et al. 2002). Potokar et al. (2013a) reported that hypoosmotic conditions affected plasma membrane localization of AQP4 in rat astrocytes, in particular hypoosmotic stimulation triggered a transient increase in AQP4 plasma membrane localization. These changes were related to changes in AQP4 vesicle traffic; an increase in AQP4 plasma membrane localization overlapped with the observed decrease in mobility of AQP4 vesicles and the subsequent decrease in AQP4 plasma membrane localization overlapped with increased AQP4 vesicle mobility. The changes in mobility occurred predominantly in directional vesicles. These studies have shown that vesicle dynamics are playing a role in the cell swelling response at least by regulating the density of AQP4 channels in the plasma membrane. Whether cell swelling can be affected by manipulating vesicle traffic remains to be studied in the future.
3.4.2 Vesicles Delivering Plasma Membrane MHC Receptors and Transporters
Intracellular traffic of astrocytic vesicles, including endosomes and lysosomes, may also serve to deliver plasma membrane-associated MHC receptors (Soos et al. 1998; Vardjan et al. 2012) and transporters, such as glutamate transporter EAAT2 (Stenovec et al. 2008) where the nature of vesicles is not clear.
On exposure to the proinflammatory cytokine, interferon-γ (IFN-γ), otherwise immunologically silent astrocytes may begin to express MHC-II molecules and antigens on their surface and act as nonprofessional antigen-presenting cells (APCs). It has been suggested that IFN-γ-activated astrocytes participate in antigen presentation and activation of CD4 helper T cells in immune-mediated disorders of the CNS including multiple sclerosis (Fontana et al. 1984; Soos et al. 1998) and experimental autoimmune encephalomyelitis (Shrikant and Benveniste 1996).
In general, the delivery of MHC-II molecules from MHC-II compartments to the cell surface of APCs is mediated via a cytoskeletal network and is most likely completed with the fusion of MHC-II-carrying late endosomes/lysosomes with the plasma membrane. Actin microfilaments (Barois et al. 1998), microtubules (Wubbolts et al. 1999; Vyas et al. 2007), and their motor proteins (Wubbolts et al. 1999; Vascotto et al. 2007) have been shown to mediate trafficking of MHC-II compartments in APCs. Only recently, the role of IFs in MHC-II trafficking was investigated in IFN-γ-activated astrocytes (Vardjan et al. 2012), which as reactive astrocytes overexpress IFs (Junyent et al. 2011).
IFN-γ was shown to induce expression of MHC-II molecules on the astrocytic plasma membrane and late endosomes/lysosomes (Vardjan et al. 2012). The latter can be specifically labeled with Alexa Fluor® 546-conjugated dextran (Fig. 3.5a) (Jaiswal et al. 2002; Gabrijel et al. 2008; Vardjan et al. 2012). Time-lapse confocal imaging and fluorescent dextran labeling of late endosomes/lysosomes in WT astrocytes and in astrocytes devoid of IFs (GFAP − / − Vim − / − ) revealed faster and more directional movement of late endosomes/lysosomes in IFN-γ-treated astrocytes than in untreated astrocytes (Potokar et al. 2013b). However, vesicle mobility was lower and less directional in IFN-γ-treated IF-deficient astrocytes than in WT astrocytes (Fig. 3.5b, c), indicating that the IFN-γ-induced increase in the mobility of MHC-II-carrying late endosomes/lysosomes is IF dependent. Application of ATP and the subsequent increase in [Ca2+]i induced attenuation of the mobility of late endosomes/lysosomes was more apparent in the presence of IFs (Fig. 3.5b, c), implying a role for IFs in this process.
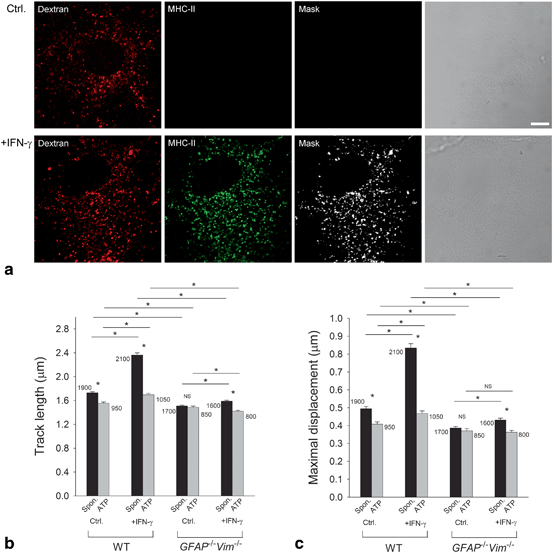
Fig. 3.5
The IFN-γ-induced increase in the mobility of MHC-II compartments in astrocytes is IF dependent. a Alexa Fluor® 546-dextran labels MHC-II-positive compartments in IFN-γ-treated WT and GFAP − / − Vim − / − (IF-deficient) primary mouse astrocytes. Fluorescence images of astrocytes labeled with dextran, fixed, and immunostained with antibodies against MHC-II molecules. White pixels (Mask) represent the colocalization mask of green (MHC-II) and red fluorescence pixels (Dextran). Scale bars: 10 µm. b Histogram of average vesicle track lengths in control (Ctrl.) and IFN-γ-treated (+IFN-γ) WT and GFAP − / − Vim − / − cells. c Histogram of the mean maximal displacements of vesicles in control (Ctrl.) and IFN-γ-treated (+IFN-γ) WT and GFAP − / − Vim − / − cells. The numbers on the bars are the numbers of vesicles analyzed. Values are mean ± SEM. *P < 0.05. (Adapted from Vardjan et al. (2012); with permission)
These data indicate that, in IFN-γ-activated astrocytes, upregulation of IFs allows faster and therefore more efficient delivery of MHC-II molecules to the cell surface. Reduced mobility of late endosomes/lysosomes due to increase in [Ca2+]i may increase their probability of docking and fusion (Potokar et al. 2010), which, in astrocytes acting as APCs, may serve as an additional regulatory mechanism that controls the onset of late endosomal/lysosomal fusion and final delivery of MHC-II molecules to the cell surface (Vardjan et al. 2012). Besides IFN-γ, endogenous suppressors, including norepinephrine, have been shown to regulate the expression of MHC-II molecules in astrocytes (Frohman et al. 1988; De Keyser et al. 2004) . The effects of norepinephrine are mediated through activation of G-protein-coupled β-ARs on astrocytes and subsequent activation of the cAMP signaling pathway. Our recent unpublished data suggest that the mobility and fusion of late endosomes/lysosomes involved in antigen presentation are also affected by activation of astrocytic β-ARs. Although these studies were carried out in vitro, all these regulatory mechanisms may enable antigen-presenting reactive astrocytes in vivo to respond rapidly and in a controlled manner during CNS inflammation.
Astrocytes play a key role in the uptake of glutamate , which is released into the extracellular space from glutamatergic neurons during synaptic transmission (Vesce et al. 1999; Amara and Fontana 2002) and from astrocytes themselves (Parpura et al. 1994). Physiologically, glutamate is cleared from the synaptic cleft via plasmalemma glial glutamate transporters GLAST (EAAT1) and GLT1 (EAAT2) (Danbolt 2001); its uptake is driven by the electrochemical gradient of sodium (O’Kane et al. 1999). The flux of transported molecules also depends on the density of transporters in the cell plasma membrane (Robinson 2002; Huang and Bergles 2004), which determines whether synaptic independence is compromised by the synaptic transmitter crosstalk. The density of EAAT2 in astrocyte plasma membrane is regulated by exo-/endocytosis in a Ca2+-dependent manner (Stenovec et al. 2008). The altered trafficking of EAAT2 to/from the plasma membrane may result in diminished net uptake of extracellular glutamate, and an overabundance of glutamate accompanied by failure of astrocytes to remove it may lead to neuronal excitotoxicity resulting in a selective loss of motor neurons as in ALS. Therefore, it may be useful to modify vesicle dynamics by drugs in order to minimize the adverse conditions associated with such a cell process.
3.5 Do Drugs Target Vesicle Dynamics in Astrocytes?
With advances in the understanding of the nature of vesicle mobility in astrocytes, a question has emerged whether there are any drugs that affect vesicle dynamics and could be used to treat neurologic diseases. Although the experimental evidence has revealed that different types of vesicles exhibit specific properties, it is possible that, under pathologic conditions, these may change. These include altered vesicle dynamics, changes in molecules that are released by vesicular mechanisms, alterations in the plasma membrane surface signaling landscape (altered densities of transporters, receptors, and associated signaling mechanisms) and changes in vesicle dynamics associated with morphology of astrocytes. All these factors may contribute to changes in communication between astrocytes and neighboring cells. However, one may consider that the least change may occur, if vesicle dynamics are attenuated, which may contribute to the minimization of vesicle-mediated changes in the cell. In line with this is the discovery that vesicle mobility was attenuated by fingolimod or FTY720 (Trkov et al. 2012). This drug was recently introduced as a therapeutic for the treatment of multiple sclerosis (Chun and Brinkmann 2011). It was shown that FTY720 accumulates in tissue hydrophobic pools (Foster et al. 2007), such as the white matter in the CNS, where it can reach concentrations that affect astrocytic vesicle mobility and consequently their ability to participate in regulated exocytosis (Trkov et al. 2012). This action may be part of its therapeutic effectiveness in patients with multiple sclerosis. The mechanism of reduction of vesicle mobility by fingolimod likely involves fingolimod-induced changes in [Ca2+]i homeostasis. Figure 3.6 shows that the application of fingolimod augments [Ca2+]i measured by the Fluo 4 fluorescent Ca2+ dye. In Fig. 3.6e, the time course of increased [Ca2+]i is shown for 5 individual astrocytes. These increases in [Ca2+]i are associated with the reduced mobility of peptidergic vesicles depicted in Fig. 3.6b–d. However, vesicle types other than peptidergic vesicles were also shown to be sensitive to fingolimod (Trkov et al. 2012). Moreover, astrocytes were considered to be the major source of a number of other molecules, including eicosanoids (prostaglandins, prostacyclins, thromboxanes, and leukotrienes) and these proinflammatory signaling molecules in the CNS are released via an ATP-dependent mechanism (Murphy et al. 1988). In astrocytes, ATP itself is released via regulated exocytosis, which participates in the neuroinflammatory and other pathologic states in the CNS. Thus, new therapeutics, such as FTY720 (Trkov et al. 2012), that affect vesicle mobility represent a novel possibility for the development of new therapeutics for neurologic diseases.
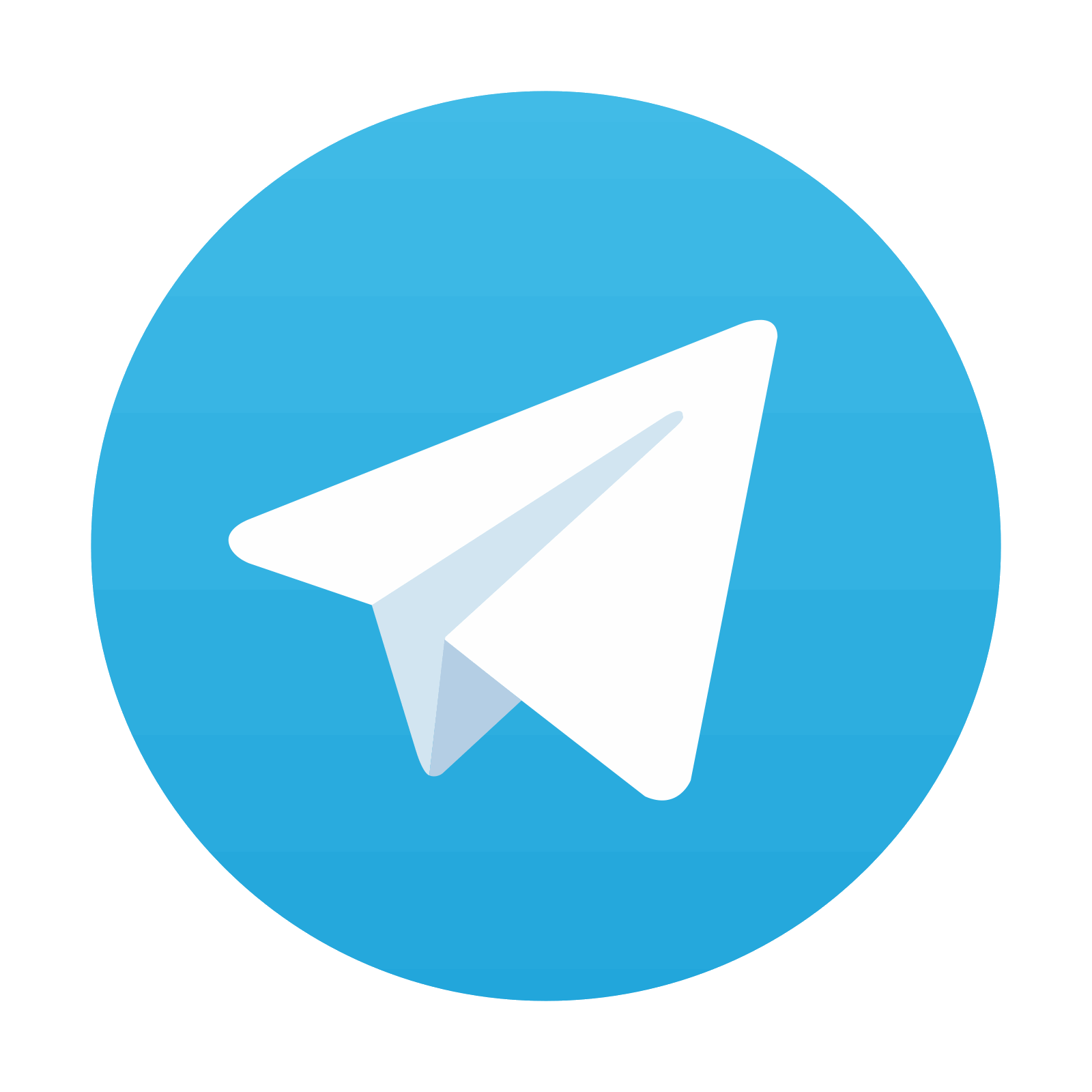
Stay updated, free articles. Join our Telegram channel
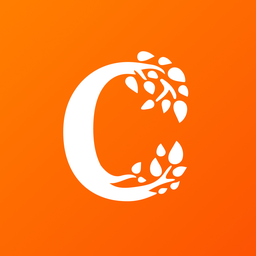
Full access? Get Clinical Tree
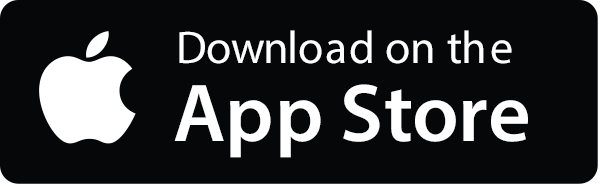
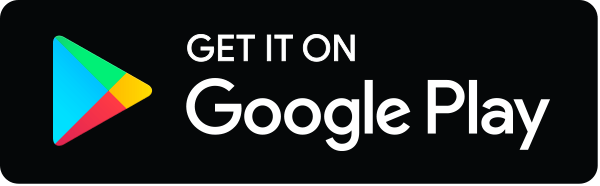