Parameters
Wakefulness
NREM sleep
REM sleep
Respiratory rate
Normal
Decreases
Variable, apnea may occur
Minute ventilation
Normal
Decreases
Decreases further
Alveolar ventilation
Normal
Decreases
Decreases further
PaCO2
Normal
Increases slightly
Increases further slightly
PaO2
Normal
Decreases slightly
Decreases further slightly
SaO2
Normal
Decreases slightly
Decreases further
Hypoxic ventilatory response
Normal
Decreases
Decreases further
Hypercapnic ventilatory response
Normal
Decreases
Decreases further
Upper airway muscle tone
Normal
Decreases slightly
Decreases markedly or is absent
Upper airway resistance
Normal
Increases
Increases further
Table 11.2
Summary of physiological changes in heart and circulation
Physiological characteristics | NREM | REM |
---|---|---|
Heart rate | ↓ | ↑↓ |
• Cardiac output | ↓ | ↓↓ |
• Systematic arterial BP | ↓ | ↓↑ |
– Dippers | ||
– Extreme dippers | ||
– Non-dippers | ||
– Reverse dippers | ||
• Pulmonary arterial BP | ↑ | ↑ |
• Peripheral vascular resistance | —↓ | ↓ |
• Systematic blood flow | ||
– Cutaneous | — | ↓ |
– Muscular | — | ↓ |
– Mesenteric | — | ↑ |
– Renal | — | ↑ |
• Cerebral blood flow | ↓ | ↑ |
Table 11.3
Physiological changes during normal wakefulness and sleep in the gastrointestinal system
Physiological characteristics | Wakefulness | Sleep |
---|---|---|
Swallowing frequency | Normal | Decreased |
Salivary flow | Normal | Decreased |
Esophageal acid clearance time | Normal | Prolonged |
Lower and upper esophageal sphincter pressure | Normal | Decreased |
Esophageal peristaltic contractions | Normal | Decreased |
Gastric motility | Normal | Decreased |
Gastric acid secretion | Depends on food ingestion | Peak Secretion between 10 p.m. and 2 a.m. |
Migrating motor complex (MMC) recurs every 90 min) | Normal velocity | Reduced velocity |
Colonic motility | Normal | Decreased |
Rectal motor activity and anal canal pressure | Normal | Increased periodic activity with retrograde propagation and higher anal canal pressure |
Changes in the Central Somatic Nervous System During Sleep
Sleep is not just a state of rest and repose; many areas of central nervous system (CNS) show excitation during sleep as demonstrated by intracellular electrophysiological studies, C-fos activation shown by immunohistochemical observations and positron emission tomographic (PET) scans of the brain. NREM sleep is characterized by enhanced activity of ventrolateral preoptic and median preoptic neurons in the anterior hypothalamic nuclei and to a certain extent also lower brainstem nucleus tractus solitarius (NTS); in contrast, the wake-promoting neurons in the ascending reticular activating system (ARAS), histaminergic, aminergic and orexinergic neurons as well as most of the cerebral cortical regions are deactivated. During REM sleep, much of the cortical regions are activated along with pontine areas controlling REM sleep coupled with a paralyzed body caused by excitation of motor atonia pathways in the brain stem and spinal cord. The combined alterations in the somatic CNS and the ANS (see further on) are responsible for vast physiological changes in the body systems and organs during sleep.
There are also changes in certain neural reflexes and cortical evoked potentials during sleep. The H-reflex, the electrical counterpart of monosynaptic muscle stretch reflex is decreased in amplitude because of motor neuron hyperpolarization, presynaptic inhibition and disfacilitation of brain stem aminergic and lateral hypothalamic orexinergic neurons projecting to brain stem motor and aminergic neurons as well as ventral horn cells of the spinal cord.
Polysynaptic reflexes which are useful in clinical studies include blink reflex and spinal flexor reflex. Certain components of these reflexes (R2) also decrease or are unobtainable during sleep. Babinski response (extensor plantar-response) which is absent in wakefulness in normal individuals may be elicited in sleep as a result of cerebral cortical inhibition and release of lower centers.
Somatosensory cortical evoked potentials (SEPs) attenuate during sleep, particularly during slow-wave sleep (SWS). Motor evoked potential (MEP) amplitude following magnetic brain stimulation also shows attenuation in all stages of sleep. There is an increased intracortical inhibition of interneurons in NREM sleep and decreased intracortical facilitation in REM sleep as determined by paired brain magnetic stimulation technique [5].
Neuroimaging studies have clearly shown areas of activation and deactivation of various regions of the brain during NREM and REM sleep, emphasizing that sleep is a variable and dynamic state and not simply a passive period in our life (see Chap. 21).
Clinical Relevance: In narcolepsy-cataplexy syndrome during cataplectic episodes, there is marked inhibition of monosynaptic reflexes including H-reflex. Blink reflex excitability study during cataplectic episodes may show facilitation of R2 component and impaired habituation suggesting involvement of brain stem interneurons in the pathogenesis of cataplectic motor phenomenon [6]. MEP amplitude during a cataplectic spell showed inconsistent results. One study documented persistence of MEP indicating enhanced cortical excitability [7], but another study [8] showed MEP amplitude attenuation. In RLS patients, spinal flexor reflex study during sleep disclosed hyperexcitability suggesting release of spinal motor centers from supraspinal inhibition [9].
Autonomic Nervous System and Sleep
Central Autonomic Network
The existence of a central autonomic network (CAN) in the brain stem with ascending and descending projections that are often reciprocally connected has been clearly shown by work done in the 1980s and 1990s (Figs. 11.1 and 11.2) [10 –11]. The NTS may be considered a central station in the CAN. The NTS, which is located in the dorsal region of the medulla ventral to the dorsal vagal nucleus, is the single most important structure of the autonomic network and is influenced by higher brain stem, diencephalon, forebrain, and neocortical regions (Fig. 11.3; see also Figs. 11.1 and 11.2). The NTS receives afferent fibers—from the cardiovascular system and the respiratory and gastrointestinal tracts—important for influencing autonomic control of cardiac rhythm and rate, circulation, respiration, and gastrointestinal motility and secretion (Fig. 11.4). Efferent projections arise from the NTS and are sent to the supramedullary structures, including hypothalamic and limbic regions, and to the ventral medulla, which exerts significant control over cardiovascular regulation [12–14]. The ventral medulla sends efferent projections to the intermediolateral neurons of the spinal cord (see Figs. 11.1 and 11.2). Box 11.1 lists afferent and efferent connections of NTS, considered a major central station of the CAN. The final common pathways from the NTS are the vagus nerve and sympathetic fibers, which send projections to the intermediolateral neurons of the spinal cord to orchestrate the CAN for integrating various autonomic functions that maintain internal homeostasis. The NTS also contains the lower brain stem hypnogenic and central respiratory neurons. Dysfunction of the ANS, therefore, may have a serious impact on human sleep and respiration.





Fig. 11.1
The ascending projections from the central autonomic network (Reprinted with permission from Chokroverty [15])

Fig. 11.2
The descending projections from the central autonomic network (Reprinted with permission from Chokroverty [15])

Fig. 11.3
Schematic diagram of central autonomic network: ascending projections from nucleus tractus solitarius (Reproduced with permission from Chokroverty [16])

Fig. 11.4
The visceral afferents to and efferents from the nucleus tractus solitarius (Reprinted with permission from Chokroverty [15])
Box 11.1. Afferent and Efferent Connections of Central Autonomic Network (CAN)
Nucleus tractus solitarius (NTS), the major central station of the CAN
Afferents
Cardiovascular
Respiratory
Taste
Gastrointestinal
Descending projections from cerebrum and upper brain stem
Efferents
Ventral medulla
Intermediolateral neurons
Inspiratory neurons
Vagal efferents to cardiovascular, respiratory and gastrointestinal systems
Ascending projections to upper brain stem and cerebrum.
The cardiovascular system and respiration play significant roles in the maintenance of the internal homeostasis in human beings [13– 17]. Cardiovascular control in humans is maintained reflexively, involving peripheral receptors in the heart and blood vessels with afferents to the CNS and efferents to the heart and blood vessels. Sympathetic preganglionic neurons regulating the cardiovascular system are located predominantly (90 %) in the intermediolateral neurons of the thoracic spinal cord, with a small number (10 %) in the adjacent spinal structures. Parasympathetic preganglionic neurons controlling the heart and circulation are located in the nucleus ambiguus as well as in the dorsal motor nucleus of the vagus in the medulla. Sympathetic preganglionic neurons in the intermediolateral column of the spinal cord as well as parasympathetic preganglionic neurons in the nucleus ambiguus and dorsal motor nucleus of the vagus are the central determinants of cardiovascular regulation. Both the sympathetic and parasympathetic preganglionic neurons have extensive connections to the CAN, which in turn is influenced by peripheral afferents (Fig. 11.5; see also Figs. 11.1, 11.2, 11.3, 11.4). There is direct projection from the hypothalamic paraventricular nucleus (PVN) to sympathetic preganglionic neurons in the spinal cord (see Figs. 11.2 and 11.5).


Fig. 11.5
Schematic diagram showing descending hypothalamic and brain stem inputs to intermediolateral neurons in the spinal cord (Reprinted with permission from Chokroverty [15])
Autonomic Changes During Sleep
During sleep in normal individuals, there are profound changes in the functions of the ANS [10, 12, 18–25] (See Box 11.2). Most of the autonomic changes that occur during sleep involve the heart, circulation, respiration, and thermal regulation. There are also pupillary changes. Pupilloconstriction occurs during NREM sleep which persists after preganglionic sympathectomy and is maintained during REM sleep due to tonic parasympathetic drive. Phasic dilation during phasic REM sleep results from central inhibition of parasympathetic outflow to the iris.
Box 11.2. Functional Changes in the AUTONOMIC NERVOUS SYSTEM DURING SLEEP
NREM Sleep
Increased parasympathetic activity
Decreased sympathetic activity
Decreased low-frequency (LF) components reflecting decreased sympathetic tone but increased high frequency (HF) components reflecting increased respiratory vagal tone
REM Sleep
Further increase in parasympathetic activity
More marked decrease in sympathetic activity
Intermittent bursts of sympathetic activity in phasic rapid eye movements
Profound increase in sympathetic activity in skin and muscle vessels (microneurographic recordings)
Variable LF and HF with decreased HF and intermittent increment of LF components.
Autonomic functions during wakefulness must be compared to those during sleep to understand ANS changes in sleep [18]. The basic ANS changes during sleep include increased parasympathetic tone and decreased sympathetic activity during NREM sleep accompanied by a reduction of circulating levels of norepinephrine and epinephrine. During REM sleep, there is further increase in parasympathetic tone and further decrease of sympathetic activity; intermittently, however, there is an increase in sympathetic activity during phasic REM sleep resulting in swings in BP and HR causing tachy–brady arrhythmias. The ANS changes during sleep can be assessed by measuring heart rate variability (HRV) [26]. The indices of HRV can be documented by fast Fourier transform showing power in the following bands: HF, LF, very low frequency (VLF), and ultra low frequency (ULF). HF band power ranges from 0.15 to 0.4 Hz. The power in the LF band ranges between 0.04 and 0.15 Hz. The VLF band power ranges from 0.003 to 0.04 Hz, and the power in the ULF band is 0.003 Hz or less. The major contributor of the HF component is the efferent vagal activity. The LF component is thought to be a marker of sympathetic modulation by some authors [27, 28], whereas others [29, 30] considered this to contain both sympathetic and vagal influences. Therefore, the LF/HF ratio is thought to reflect sympathovagal balance [28, 29]. The significance of VLF and ULF components remains uncertain, but these may be markers of humoral and hormonal fluctuations. Both spectral components and direct nerve recordings (see below) show that the HF component predominates during NREM and the LF component predominates during REM sleep. During NREM sleep, the LF component decreases, whereas the HF component increases, reflecting increased vagal tone. In contrast, during REM sleep, extreme variation in LF and HF with increased LF and decreased HF components is noted. The heart rate (HR) changes precede electroencephalographic (EEG) changes during transition of sleep states. HRV is similar in presleep and intrasleep wake periods. It should be further noted that the HF component mainly reflects the respiration–vagal modulation of sinus rhythm, whereas the nonrespiratory LF component reflects the sympathetic modulation of the heart in addition to baroreflex responsiveness to beat-to-beat variations in blood pressure (BP) [31,32]. Power spectrum analysis of normal subjects at sleep onset by Shiner et al. [33] showed that the wake/sleep transition period represents a transitional process between two physiologically different states, with a decrement of LF power and unchanged HF power causing a decrement of the LF/HF ratio reflecting a shift toward parasympathetic predominance. Thus, NREM sleep can be considered as a state of relative cardiorespiratory stability, whereas REM sleep is a state of profound instability with an intense autonomic and respiratory dysregulation. In a recent study, Richard et al. [34] pointed to the effect of gender on autonomic and respiratory responses during sleep. They noted that, in women, there was a greater NREM-to-REM increment in LF, a greater decrement in HF, and a greater increment in LF/HF power. NREM-to-REM excitatory cardiorespiratory responses are, therefore, more marked among women compared to men.
There is also a profound change of sympathetic activity in muscle and skin blood vessels. Microneurographic technique measures peripheral sympathetic nerve activity in the muscle and skin vascular beds. The technique permits direct intraneural recording of efferent sympathetic nerve activity involving the muscle and skin blood vessels by using tungsten microelectrodes [35–39]. Muscle sympathetic nerve activity is reduced by 10–30 % in stages N1 and N2 sleep and by 30–50 % from wakefulness to SWS, but increases to levels above waking values during REM sleep [38]. Although sympathetic nerve activity increases in the skeletal muscle vessels (vasoconstriction) during REM sleep, the sympathetic drive decreases in the splanchnic and renal circulation (vasodilation) [38]. Sympathetic nerve activity is lower during NREM sleep than during wakefulness but increases above the waking level during REM sleep, particularly during phasic REM sleep (Fig. 11.6). During the arousal and appearance of K complexes in NREM sleep, the bursts of sympathetic activity transiently increase.


Fig. 11.6
Sympathetic nerve activity (SNA) during wakefulness (W), NREM sleep (Stages N1 an N2) and REM sleep (Stage R) in a normal subject shown schematically. Progressive decrement of SNA from W to Stage N2 and Stage N3 but marked increment in Stage R (Adapted from: Somers et al. [38])
Clinical Relevance
The implications of changes in the ANS during sleep in humans are profound. Reduced HRV may be noted in patients with myocardial infarction, cardiac transplantation, and diabetic autonomic neuropathy [26, 27, 30, 40, 41]. There is a significant relationship between the ANS and cardiovascular mortality, including sudden cardiac death. Lethal arrhythmias are related to either increased sympathetic activity or decreased vagal activity. HRV (e.g., reduced HRV) is a strong and independent predictor of mortality after acute myocardial infarction. HRV study thus has potential for assessment of ANS fluctuations in patients with cardiovascular and noncardiovascular disorders and may help us understand physiological phenomena, disease mechanisms, and effects of medications. Furthermore, disorders of the ANS in humans, such as multiple systems atrophy, familial dysautonomia, and secondary autonomic failure (see Chap. 41), adversely affect respiratory and cardiovascular functions during sleep. A number of human sleep disorders may affect autonomic functions (e.g., obstructive sleep apnea syndrome (OSAS), cluster headache, sleep terrors, REM sleep behavior disorder and narcolepsy [25, 42, 43]). Thus, significant sleep-related changes in the ANS affecting the circulatory, respiratory, gastrointestinal, and urogenital systems have important clinical implications in patients with central or peripheral autonomic failure (e.g., sleep-related respiratory dysrhythmias, cardiac arrhythmias, gastrointestinal dysmotility and urogenital disorders). Thus, there is a bidirectional relationship between the ANS and sleep: autonomic changes can alter sleep regulation and in turn sleep disturbances can affect the ANS.
In patients with OSAS, chronic sympathetic hyperactivity is thought to play a major role in linking OSAS with hypertension, cardiac ischemia, congestive cardiac failure and stroke [41, 42]. A variety of cardiovascular reflex tests measuring HR and BP changes to special maneuvers (e.g., head-up tilt, valsalva maneuver, deep breathing, hand grip, cold face, cold pressor and mental arithmetic tests) can detect integrity of sympathetic and parasympathetic divisions of the ANS as well as baroreflex [23, 43]. Baroreflex is an important mechanism to maintain BP homeostasis [22–25]. Baroreflex function [44, 45] can be evaluated by an indirect technique of baroreflex sensitivity (BRS) which is defined as change in HR per unit change in arterial BP [23, 24]. The slope of the changes in RR interval (or HR) in relation to BP changes determines BRS which indirectly assesses baroreflex function. BRS is increased during NREM sleep as result of resetting of baroreflex function, and this results in slowing of the HR despite a decrement of BP [22, 23, 43, 45].
Other tests to evaluate ANS function included HR spectral analysis of HRV and recording of muscle and skin sympathetic nerve activity (MSNA and SSNA) using tungsten microelectrodes inserted into the peroneal and median nerves [38]. Additionally, imaging studies are available such as MIBG scintigraphy [46] to assess cardiac sympathetic innervation, which is impaired in post ganglionic cardiovascular autonomic disorders.
Respiration and Sleep
Functional Neuroanatomy of Respiration
In order to understand the control of breathing, it is essential to have a basic knowledge about the functions of breathing, the functional anatomy of breath, and control of respiration during wakefulness and asleep. The function of breathing is to maintain an adequate alveolar ventilation and diffusion across the alveolar capillary membranes (i.e., elimination of carbon dioxide [CO2] to and supply of oxygen [O2] from the atmospheric air containing 21 % O2 78 % nitrogen, and 1 % other inert gases), and to maintain arteriolar homeostasis (i.e., normal partial pressure of oxygen [PO2] and carbon dioxide [PCO2]). An adequate pulmonary circulation is essential to complete the processes of alveolar ventilation and diffusion. The respiratory system consists of three interrelated and integrated components: central controllers located in the medulla aided by the supramedullary structures, including forebrain, peripheral chemoreceptors, and pulmonary and upper airway receptors; the thoracic bellows, consisting of respiratory and other thoracic muscles and their innervation and bones; and the lungs, including the airways.
In 1812, Legallois [47] discovered that breathing depends on a circumscribed region of the medulla. After an intensive period of research in the nineteenth century on the respiratory centers, in the twentieth century, Lumsden [48, 49], and later Pitts et al. [50], laid the foundation for modern concepts of the central respiratory neuronal networks. Based on sectioning at different levels of the brain stem of cats, Lumsden [48, 49] proposed pneumotaxic and apneustic centers in the pons and expiratory and gasping centers in the medulla. Later, Pitts’ group [50] concluded from experiments with cats that the inspiratory and expiratory centers were located in the medullary reticular formation.
Upper brain stem respiratory neurons are located in the rostral pons, in the region of the parabrachial and Kölliker-Fuse nuclei (pneumotaxic center), and in the dorsolateral region of the lower pons (apneustic center) [51]. These two centers influence the automatic medullary respiratory neurons, which comprise two principal groups [51–59]. The dorsal respiratory group (DRG) located in the NTS is responsible principally, but not exclusively, for inspiration, and the ventral respiratory group (VRG) located in the region of the nucleus ambiguus and retroambigualis is responsible for both inspiration and expiration (Fig. 11.7). The VRG contains the Botzinger complex in the rostral region and the pre-Botzinger region immediately below the Botzinger complex, responsible mainly for the automatic respiratory rhythmicity as these neurons have intrinsic pacemaker activity. These respiratory premotor neurons in the DRG and VRG send axons that decussate below the obex and descend in the reticulospinal tracts in the ventrolateral cervical spinal cord to form synapses with the spinal respiratory motor neurons innervating the various respiratory muscles (see Figs. 11.3 and 11.4). Respiratory central pattern generator (RPG) [60] includes neurons in the dorsolateral pons (e.g., parabrachial [PB], and Kolliker-Fuse [KF] nuclei in this region), NTS and ventrolateral medulla (Ventral respiratory group [VRG]). Box 11.3 summarizes functions of various respiratory neurons [60]. The site of central chemoreceptor zone is not definitely known but most likely located in the retrotrapezoid nucleus (RTN) in the rostral medulla (part of the parafacial nucleus) between the facial nucleus in the lower pons and the ventral surface of the medulla, and contains glutamatergic neurons [61, 62]. RTN neurons innervate also the brainstem area containing the RPG [61]. The neurons in the arcuate nucleus at the ventromedial aspect of the medullary pyramid may also have a role in central chemosensitivity and respiratory frequency [60].


Fig. 11.7
Schematic representation of central respiratory neurons in Pons and Medulla. DRG Dorsal respiratory group with projections to contralateral predominantly inspiratory muscles. VRG Ventral respiratory group with projections to contralateral inspiratory and expiratory muscles. P Pneumotaxic center; A Apneustic center. See text for further details
Box 11.3. Functions of Respiratory neurons [60]
PB and KF nuclei control the phase-switch between inspiration and expiration.
The NTS is the primary relay station for medullary reflexes controlling respiration.
The Botzinger complex (in the VRG) contains expiratory neurons.
The pre-Botzinger complex is responsive for respiratory rhythm generation.
The rostral VRG contains inspiratory neurons.
The caudal VRG contains expiratory neurons.
The DRG contains mainly, but not exclusively inspiratory neurons
PB: parabrachial; KF: Kölliker-Fuse
NTS: nucleus tractus solitarius
VRG: ventral respiratory group
DRG: dorsal respiratory group.
Control of breathing by the medullary respiratory neurons to regulate the rate, rhythm, amplitude, rhythmicity and pattern of breathing, and internal homeostasis depends on a variety of afferent inputs to respiratory neurons in the brainstem. These include the parasympathetic vagal afferents from the peripheral respiratory tracts and receptors, the carotid and aortic body peripheral chemoreceptors (sensing predominantly low O2 but to an extent also increased CO2 and H+), the central chemoreceptors in the medulla sensing alteration in CO2 or H(+) in the local circulation, the supramedullary structures (e.g., forebrain, midbrain, and pontine regions), and the reticular activating systems [51, 63–66].
Breathing is controlled during wakefulness and sleep by two separate and independent systems [52–57, 67]: the metabolic or automatic [53, 54], and the voluntary or behavioral [67]. Both metabolic and voluntary systems operate during wakefulness, but breathing during sleep is entirely dependent on the inherent rhythmicity of the autonomic (automatic) respiratory control system located in the medulla [55–57]. Voluntary control is mediated through the behavioral system that influences ventilation during wakefulness as well as nonrespiratory functions [68, 69] such as phonation and speech. In addition, the wakefulness stimulus, which is probably derived from the ARAS [70–72], exerts a tonic influence on the brain stem respiratory neurons during wakefulness.
The voluntary control system for breathing originating in the cerebral cortex (forebrain and limbic system) controls respiration during wakefulness and has some nonrespiratory functions [65, 67]. This system descends with the corticobulbar and corticospinal tracts partly to the automatic medullary controlling system and to some degree both terminates and integrates there. However, it primarily descends with the corticospinal tract to the spinal respiratory motor neurons, in the high cervical spinal cord, where the fibers finally integrate with the reticulospinal fibers originating from the automatic medullary respiratory neurons for smooth, coordinated functioning of respiration during wakefulness [53, 54, 57, 73, 74].
There is a close interrelationship between the respiratory [52–54] central autonomic [12, 75, 76], and lower brain stem hypnogenic neurons [77–83] in the pontomedullary region. The hypothalamic and lower brain stem hypnogenic neurons are also connected [84]. Reciprocal connections exist between the hypothalamus, the central nucleus of the amygdala, parabrachial and Kölliker-Fuse nuclei, and the NTS of the medulla (see Figs. 11.1 and 11.2) [75, 11, 73, 15]. In addition, the NTS connects with the nucleus ambiguus and retroambigualis (see Fig. 11.2) [75, 11, 73, 15]. Thus, their anatomic relationships suggest close functional interdependence among the CAN, the respiratory and hypnogenic neurons. In addition, peripheral respiratory receptors (arising from the pulmonary and tracheobronchial tree) and chemoreceptors (peripheral and central) interact with the CAN in the region of the NTS [51–54, 85].
The thoracic bellows component consists of thoracic bones, connective tissue, pleural membranes, the intercostal and other respiratory muscles, and the nerves and blood vessels. Respiratory muscle weakness plays a critical role in causing sleep dysfunction and sleep-disordered breathing in neuromuscular disorders. Box 11.4 lists the respiratory muscles. The main inspiratory muscle is the diaphragm (innervated by the phrenic nerve, formed by motor roots of C3, C4, and C5 anterior horn cells), assisted by the external intercostal muscles (innervated by the thoracic motor roots and nerves), which expand the core of the thoracic cavity and lungs during quiet normal breathing. Expiration is passive, resulting from elastic recoil of the lungs. During forced and effortful breathing (e.g., dyspnea and orthopnea), accessory muscles of respiration assist the breathing. Accessory inspiratory muscles include the sternocleidomastoideus, trapezius, and scalenus (anterior, middle, and posterior) as well as the pectoralis, serratus anterior, and latissimus dorsi. Accessory expiratory muscles consist of internal intercostal and abdominal muscles (e.g., rectus abdominis, external and internal oblique, and transversus abdominis) innervated by thoracic motor roots and nerves. Normally, these three respiratory components (central controllers, chest bellows, and lungs) function smoothly in an automatic manner to permit gas exchange (transfer of O2 into the blood and elimination of CO2 into the atmosphere) for ventilation, diffusion, and perfusion. Minute ventilation is defined as the amount of air breathed per minute, which equals about 6 L; about 2 L stay in the anatomic dead space, consisting of the upper airway and the mouth, and 4 L participate in gas exchange in the millions of alveoli constituting alveolar ventilation. Respiratory failure may occur as a result of dysfunction anywhere within these three major components of the respiratory control systems.
Box 11.4 The Respiratory Muscles
Inspiratory Muscles
Diaphragm
External intercostal
Accessory Inspiratory Muscles
Sternocleidomastoideus
Scalenus (anterior, middle, posterior)
Pectoralis major
Pectoralis minor
Serratus anterior
Serratus posterior superior
Latissimus dorsi
Alae nasi
Trapezius.
Expiratory Muscles (silent during quiet breathing but contract during moderately severe airway obstruction or during forceful and increased rate of breathing)
Internal intercostal
Rectus abdominis
External and internal oblique
Transversus abdominis.
Control of Ventilation During Wakefulness
The function of ventilation is to maintain arterial homeostasis (i.e., normal partial pressure of oxygen [PO2] and carbon dioxide [PCO2]) [86]. The PCO2 depends predominately on the central chemoreceptors with some influence from the peripheral chemoreceptors, whereas PO2 depends entirely on the peripheral chemoreceptors. To maintain optimal PO2 and PCO2 levels, the metabolic or autonomic respiratory system uses primarily the peripheral and central chemoreceptors but also to some extent the body’s metabolism and the intrapulmonary receptors [86]. It is well known that hypoxia and hypercapnia stimulate breathing [87, 88]. Hypoxic ventilatory response is mediated through the carotid body chemoreceptors [89, 90]. Normally, this response represents a hyperbolic curve that shows a sudden increase in ventilation when PO2 falls below 60 mm Hg [51, 55–57, 86] (Fig. 11.8). Conversely, the hypercapnic [86, 88] ventilatory response is linear (see Fig. 11.8). It is mediated mainly through the medullary chemoreceptors [91] but also to some extent through the carotid body peripheral chemoreceptors [89]. The central respiratory chemosensitivity depends predominantly on an adequate response of chemosensory neurons of the RTN to sensing changes in CO2 or H(+) in the local circulation [92]. When PCO2 falls below a certain minimum level, which is called the apnea threshold, ventilation is inhibited [86]. The metabolic rate (e.g., carbon dioxide production [VCO2] or oxygen consumption [VO2], particularly VCO2) affects ventilation in part [86]. During sleep, metabolism slows. The intrapulmonary receptors do not seem to play a major role in normal human ventilation [86]. The Hering-Breuer reflex, important to respiration, depends on pulmonary stretch receptors. Vagal afferent stimulation by increasing lung inflation terminates inspiration.


Fig. 11.8
Hypoxic and hypercapnic ventilatory responses during wakefulness, NREM (Stages N1, N2 and N3) and REM (Stage R) sleep in a normal man shown schematically. There is progressive decrement of responses with shift to the right from wakefulness to Stages N1, N2, N3 and REM state (Stage R). (Adapted from References [93, 94]
Control of Ventilation During Sleep
Changes in Ventilation
During NREM sleep, minute ventilation falls by approximately 0.5–1.5 L/min [86, 92, 96, 97, 101, 102, 103, 104] and this is secondary to reduction in the tidal volume. REM sleep shows a similar reduction of minute ventilation, up to approximately 1.6 L/min [86, 92, 98, 102, 104, 105]. Although there is a discrepancy in the literature regarding REM sleep-related ventilation in humans, it is generally accepted that most reduction occurs during phasic REM sleep.
The following factors, in combination, may be responsible for alveolar hypoventilation during sleep [86, 92]: reduction of VCO2 and VO2 during sleep, absence of the tonic influence of the brain stem reticular formation (i.e., absence of the wakefulness stimulus), reduced chemosensitivity (see Chemosensitivity and Sleep later), and increased upper airway resistance to airflow resulting from reduced activity of the pharyngeal dilator muscles during sleep [102, 106].
Changes in Blood Gases
As a result of the fall of alveolar ventilation, the PCO2 rises by 2–8 mm Hg, PO2 decreases by 3–10 mm Hg, and arterial oxygen saturation (s) decreases by less than 2 % during sleep [96, 98, 99, 107, 108]. These changes occur despite reductions of VO2 and VCO2 during sleep [109].
Respiratory Rate and Rhythm
In NREM sleep, the respiratory rate primarily shows a slight decrement, whereas in REM sleep the respiration becomes irregular, especially during phasic REM [86]. There is also waxing and waning of the tidal volume during sleep onset resembling Cheyne-Stokes breathing [95, 98, 109–111] which is related to several factors [86]: sudden loss of wakefulness stimulus, reduced chemosensitivity at sleep onset (see Chemosensitivity and Sleep later), and transient arousal. During the deepening stage of NREM sleep, respiration becomes stable and rhythmic and depends entirely on the metabolic controlling system [55–57, 65, 86, 98].
Chemosensitivity and Sleep
A rising arterial PCO2 and falling arterial PO2 stimulate ventilation. Hypoxia acts through peripheral chemoreceptors in the carotid bodies, whereas CO2 acts mainly through the central chemoreceptor in the medulla and also to an extent through the carotid body chemoreceptor.
Hypoxic ventilatory response in humans is decreased in NREM sleep in adult men but not in women, whereas hypoxic ventilatory response during REM sleep is significantly decreased in both men and women (Fig. 11.8) [93, 95, 112–116]. The underlying mechanisms for this gender difference are not clear [117]. This reduction could result from two factors: (1) increased upper airway resistance to airflow during all stages of sleep [102, 106, 118] and (2) decreased chemosensitivity.
Hypercapnic ventilatory response also decreases by approximately 20–50 % during NREM sleep [96, 99, 103, 94, 119] and further during REM sleep (Fig. 11.8) [116, 94, 119]. This results from a combination of two factors: (1) a decreased number of functional medullary respiratory neurons during sleep and (2) increased upper airway resistance [102, 106, 118]. During sleep, the CO2 response curve shifts to the right so that increasing amounts of PCO2 are needed to stimulate ventilation [108]. These findings suggest decreased sensitivity of the central chemoreceptors subserving medullary respiratory neurons during sleep. The marked blunting of the hypercapnic ventilatory response during REM sleep could also be related to increasing brain blood flow during this sleep state [107].
Metabolism and Ventilation During Sleep
There is a definite decrease in VCO2 and VO2 during sleep [109, 120, 121]. Metabolism slows suddenly at sleep onset and accelerates slowly in the early morning at approximately 5:00 a.m. [109]. During sleep, ventilation falls parallel to metabolism. The rise of PCO2 during sleep, however, is due to alveolar hypoventilation and is not related to reduced metabolism [86]. The role of the intrapulmonary receptors during normal sleep in humans is unknown [86].
Arousal Responses During Sleep
Hypercapnia is a stronger arousal stimulus than hypoxemia during sleep. An increase in PCO2 of 6–15 mm Hg causes consistent arousal during sleep [113], whereas SaO2 would have to decrease to 75 % before arousing a normal person [106, 122].
Laryngeal stimulation normally causes cough reflex response, but this is decreased during both states of sleep and is more markedly decreased during REM than NREM sleep [123]. Thus, clearance of aspirated gastric contents is impaired during sleep. In infants, laryngeal stimulation causes OSA, and this has been postulated as one mechanism for sudden infant death syndrome (SIDS) [124].
Upper Airway Reflexes, Receptors and Resistance
The upper airway reflexes are important in controlling the upper airway resistance and upper airway space. The activity of the upper airway inspiratory dilator muscles reflexively increases at the onset of inspiration due to the negative intrathoracic pressure. These respiratory-related increased pharyngeal muscle activations during inspiration are an attempt to enlarge the upper airway space to resist the sub-atmospheric inspiratory airway collapsing pressure. These upper airway muscles can be considered, in a way, respiratory muscles (“secondary” respiratory muscles) which modulate rather than generate airflow (see Table 11.4). Such reflex responses, however, are decreased during sleep, making the upper airway susceptible to suction collapse. This decreased upper airway reflex response probably results from decrement in the excitability of the upper airway motor neurons. The pressure sensitive upper airway muscle receptors send impulses via the glossopharyngeal (IXth cranial) and vagal (Xth cranial) nerves to respiratory centers in the medulla. The role of the upper airway reflexes in maintaining the upper airway resistance is strengthened by the observation of increased frequency of obstructive apneas and hypopneas in normal subjects during sleep following upper airway anesthesia [125] as well as increased apnea index after upper airway anesthesia in snorers [126]. Patients with OSA, however, do not show an increase in their apnea index after upper airway anesthesia which does not support a role for upper airway reflexes in the pathophysiology of OSAS [127, 128]. Alcohol, benzodiazepines, and age clearly cause a decrement in upper airway reflex response [129–131]. There are two other receptors important for control of ventilation: intrapulmonary stretch receptors sending afferent impulses via the vagus (Xth cranial) nerve to the inspiratory neurons in the NTS in the medulla responsible for reflex termination of inspiration (Hering-Breuer reflex); and lung parenchymal irritant or J receptors causing increased ventilatory effort associated with pulmonary congestion.
Table 11.4
Upper airway dilator muscles (secondary respiratory muscles)
Muscles | Innervation |
---|---|
Nasal muscles | |
• Compressor naris • Dilator naris • Alae nasi | VII VII VII |
Palatal muscles | |
• Palatoglossus • Palatopharyngeus • Levator veli palatini • Tensor veli palatini • Musculus uvulae | X X X V X |
Tongue muscles | |
• Protruders – Genioglossus – Geniohyoid • Retractors – Hyoglossus – Styloglossus – Palatoglossus | XII C1–C2 (carried by XII) XII XII XII |
Pharyngeal muscle (constrictors) | |
• Superior pharyngeal • Middle pharyngeal • Interior pharyngeal • Stylopharyngeus | X X X IX |
Muscles of mastication (control position of the mandible and hyoid bone opening the pharyngeal airway) | |
• Temporalis • Masseter • Lateral pterygoids • Medial pterygoids | V V V V |
Hyoid muscles | |
• Suprahyoid – Mylohyoid – Hyoglossus – Digastric (anterior belly) – Digastric (posterior belly) – Stylohyoid – Geniohyoid • Infrahyoid – Sternohyoid – Omohyoid – Sternothyroid – Thyrohyoid • Laryngeal muscles – Posterior cricoarytenoid – Lateral cricoarytenoid – Interarytenoid – Thyroarytenoid – Cricothyroid – Aryepiglottic – Thyroepiglottic | V XII V VII VII C1–C2 (carried by XII) CI–C4 C1–C4 C1–C4 C1–C4 X X X X X X X |
Changes in the Upper Airway and in Intercostal Muscles and Diaphragm Tone
Upper airway resistance increases during sleep as a result of hypotonia of the upper airway dilator muscles [100, 102, 106, 118, 132, 133] (see Physiologic Changes in the Neuromuscular System later). There is also hypotonia of the intercostal muscles and atonia during REM sleep. The phasic activities in the diaphragm are maintained, but the tonic activity is reduced during REM sleep [57]. As a result of the supine position and hypotonia of intercostal muscles, the functional residual capacity (FRC) decreases [134, 135]. In most normal individuals, there are circadian changes in airway patency with mild bronchoconstriction during sleep at night [136].
Summary and Conclusions
During wakefulness, both metabolic and voluntary (behavioral) control systems are active. In NREM sleep, the voluntary system is inactive and respiration is entirely dependent on the metabolic controller—behavioral influences and wakefulness stimuli are not controlling respiration. The nature of ventilatory control during REM sleep has not been determined definitively, but most likely the behavioral mechanism is responsible for controlling breathing in REM sleep. Ventilation is unstable during stage N1, stage N2 and REM sleep, and apneas may occur, particularly at sleep onset and during REM sleep. Respiratory homeostasis is thus relatively unprotected during sleep. However, the breathing is more regular and stable during stage N3 (SWS). Following three mechanisms may be responsible for this singly or in combination:
- 1.
Increased genioglossus muscle single motor unit (SMU) activity during SWS compared with stage N1 or stage N2 [137]. This may be related to three possible factors: (a) higher PCO2 during SWS than stage N2 (this is unlikely but possible); (b) higher upper airway resistance during stage N3 than stages N1 and N2 triggering activation of local mechanoreceptors (variable results in prior studies); and (c) increased central respiratory drive (a theoretical possibility as no prior study is available to prove this).
- 2.
Increased arousal threshold in SWS compared with stages N1 or N2, preventing arousal related instability of breathing [138].
- 3.
Increased phasic upper airway muscle activity in SWS [139].
The major cause of hypoventilation and reduced ventilatory response to chemical stimuli during sleep is increased airway resistance [102, 106, 118, 140]. The increased resistance results from reduced activity of the pharyngeal dilator muscles as well as decreased output from the sleep-related medullary respiratory neurons [141]. The reduction of the medullary respiratory neuronal activity in sleep causes a loss of the tonic and phasic motor output to the upper airway muscles, resulting in an increase in airway resistance. Other factors that contribute to sleep-related hypoventilation include the following [55–57, 65, 86, 142]: reduction of metabolic rate by approximately 10–15 %; absence of wakefulness stimuli; reduced chemosensitivity; increased blood flow to the brain during REM sleep, which may depress central chemoreceptor activity; and functional alterations in the CNS during sleep, such as cerebral cortical suppression due to reticular inhibition and physiological cortical deafferentation (presynaptic and postsynaptic inhibition of the afferent neurons [143]) as well as postsynaptic inhibition of motor neurons during REM sleep (see Physiologic Changes in the Neuromuscular System later).
Physiological Changes in the Neuromuscular System
Physiological changes have been noted during sleep in both the somatic nervous system and the ANS that in turn produce changes in the somatic and smooth muscles of the body. This section presents a discussion of the physiological changes noted during sleep in the somatic muscles, including cranial, limb, and respiratory muscles.
Changes in Limb and Cranial Muscles
Alterations of limb and cranial muscle tone are noted during sleep. Muscle tone is maximal during wakefulness, slightly decreased in NREM sleep, and markedly decreased or absent in REM sleep. Electromyography (EMG), particularly of the mentalis or the submental muscles, is necessary to identify REM sleep and is thus important for scoring technique. In addition, transient phasic (myoclonic) bursts are noted during REM sleep. An important EMG characteristic is documentation of periodic limb movements in sleep, which are noted in the majority of patients with restless legs syndrome; patients with a variety of sleep disorders; and normal individuals, most commonly elderly ones.
Upper Airway Muscles and Sleep
Changes occur in the function of the upper airway dilator muscles (Table 11.4) during sleep that have important clinical implications, particularly for patients with sleep apnea syndrome. The upper airway dilator muscles can be considered secondary respiratory muscles which are responsible for modulating and maintaining airflow but not for generating airflow and controlling breathing [144]. These muscles are located in the nose, tongue, soft palate, pharynx, hyoid arch and larynx (Table 11.4). All these upper airway muscles show phasic bursts synchronous with inspiratory intercostal muscles (external intercostal and diaphragmatic activities). The coordinated activity of these upper airway dilator muscles by generating opposing forces to counter the collapse of upper airway (resulting from negative subatmospheric pharyngeal pressure induced by diaphragmatic contraction) maintains upper airway patency [130, 131, 144] for a smooth performance of normal breathing. The upper respiratory tract subserves both respiratory and nonrespiratory functions [145]. In experimental studies in cats, pharyngeal motor neurons in the vagus and glossopharyngeal nerves were found to be located in the medulla, overlapping the medullary respiratory neurons [146]. The experimental study by Bianchi et al. [147] demonstrated that, after changes induced by chemical stimuli (normocapnic hypoxia and normoxic hypercapnia), pharyngeal motor activities are more sensitive than phrenic nerve activation. The influence of sleep on respiratory muscle function has been reviewed by Gothe et al. [148] and Horner [130, 131].
Nasal Muscles
The compressor and dilator naris, and alae nasi muscles are the most rostral part of the upper airway secondary respiratory muscles. EMG recordings have been shown that the phasic inspiratory activation of the alae nasi muscles precedes the onset of airflow and diaphragmatic activation during wakefulness and sleep, and this interval is increased during NREM sleep [144]. This asynchrony in susceptible individuals may contribute to upper airway collapse during sleep [144]. The phasic alae nasi inspiratory activity is maintained, although decreased, during apneic episodes in OSA. Nasal CPAP is shown to reduce EMG muscle tone in the alae nasi suggesting that nasal CPAP may open the upper airway passively [127].
Genioglossus Muscle
This is the principal tongue muscle and has been studied best to show its role in OSA. The genioglossus and geniohyoid muscles are mainly responsible for forward displacement of the tongue (protrusion), whereas three other tongue muscles (Table 11.4) are responsible for backward displacement of the tongue (retrusion). EMG recordings can be obtained by intramuscular electrodes percutaneously via a submental or transoral approach or by submental surface electrodes which may record additional activation from the neighboring muscle (e.g., mylohyoid, anterior belly of the digastric, hyoglossus and geniohyoid) [144]. Genioglossal EMG activities consist of phasic inspiratory bursts and variable tonic discharges [137, 139, 144], which are mildly decreased during NREM sleep but markedly decreased during REM sleep [144–153]. In OSA patients, this sleep-dependent hypotonia of the tongue may be responsible for backward displacement of the tongue partially or completely against the posterior pharyngeal wall causing apnea or hypopnea in an anatomically compromised posterior airway. Thus, the genioglossus muscle plays an important role in upper airway OSAS. In OSA patients, genioglossal muscle tone is increased during wakefulness as a compensatory measure.
Selective reduction of genioglossal or hypoglossal nerve activity (i.e., disproportionately more reduction than the diaphragmatic or phrenic activities) has been noted with alcohol, diazepam, and many anesthetic agents [149]. Conversely, protriptyline and strychnine selectively increase such activity [150].
Palatal Muscles
Levator veli palatini and palatoglossus muscles in humans show phasic inspiratory and tonic expiratory activities [154, 155], but tensor veli palatini muscle shows tonic activity during both inspiration and expiration in wakefulness and sleep [156, 157]. During sleep in normal individuals, palatal muscles (palatoglossus, tensor veli palatini, and levator veli palatini) show decreased tone causing increased upper airway resistance and decreased airway space.
Masseter Muscle
Masseter contraction closes the jaw and elevates the mandible. In sleep apnea patients, masseter activation is present during eupneic episodes but decreased during apneic ones. Masseter EMG activity decreases immediately before the apnea, is absent during the early part of the episode, and increases at the end of the apneic period [158]. Based on experiments using chemical stimuli, Suratt and Hollowell [158] concluded that masseter activity can be increased by hyperoxic hypercapnia and inspiratory resistance loading. It appears that phasic EMG bursts start in the masseter at the same time as in the genioglossus and the diaphragm. Suratt and Hollowell [158] did not find phasic activity in masseter muscle in normal subjects during regular breathing, but noted such activity during inspiratory stimulation such as inspiratory resistance loading or hypercapnia. In sleep apnea patients, spontaneous phasic masseter activity was noted during regular breathing. Using surface electrodes, the present author has documented phasic respiratory bursts in masseter muscle in normal subjects and those without sleep apnea (Fig. 11.9) [unpublished observation].


Fig. 11.9
A 120-s epoch from polysomnogram of a year-old healthy subject showing muscle bursts in the surface electromyographic (EMG) recording from right (R) masseter muscle during stage N2. EMG bursts are seen during both expiratory (green arrows) and inspiratory (purple arrow) phases but more frequently seen during expiration. Top six channels Electroencephalogram (international nomenclature); E1-M2 and E2-M2 Left and right electro-oculogram; Lt O ocu left orbicularis oculi; EKG Electrocardiogram; Hr Heart rate; Oral Nas Oronasal thermistor PFlow Nasal pressure transducer, chest and Abd Chest and abdominal respiratory effort channels; R Interco right external intercostal (inspiratory) EMG channel; R masse right masseter muscle; SaO2 arterial oxygen saturation by finger oximetry; Chin 1-Chin 2 submental EMG; R sterno right sternocleidomastoideus EMG; Rt FCR right flexor carpi radialis EMG; Rt EDC right extensor digitorum communis EMG; Lt FCR left flexor carpi radialis EMG; Rt Quad right quadriceps EMG; Lt Quad left quadriceps; Rt Tib right tibialis anterior EMG; Rt Gast right gastrocnemius EMG; Lt Tib Left tibialis anterior EMG; Lt Gast left gastrocnemius EMG
Intrinsic Laryngeal Muscle Activity
Intrinsic laryngeal muscles, controlled by the brain stem neuronal mechanism, play an important role in the regulation of breathing [159–161]. In addition, the larynx participates in phonation, deglutition, and airway protection [160]. The posterior cricoarytenoid (PCA) muscle is the main vocal cord abductor. Laryngeal EMG can be performed by placing hooked wire electrodes percutaneously through the cricothyroid membrane [161].
PCA demonstrates phasic inspiratory bursts in normal subjects during wakefulness and NREM sleep [159]. In addition, there is tonic expiratory activity in wakefulness that disappears with NREM sleep. In REM sleep, PCA EMG shows fragmented inspiratory bursts and variable expiratory activity. During isocapnic hypoxia and hyperoxic hypercapnia, normal subjects show increased phasic inspiratory PCA activity but minimal increase in tonic expiratory activity [159].
Hyoid Muscles
Suprahyoid muscles (those inserted superiorly on the hyoid bone) include the geniohyoid, mylohyoid, hyoglossus, stylohyoid, and digastric muscles [162]. Infrahyoid muscles (those that insert inferiorly) include the sternohyoid, omohyoid, sternothyroid and thyrohyoid muscles [162]. The size and shape of the upper airways can be altered by movements of the hyoid bone. Motor neurons supplying these muscles are located in the pons, the medulla, and the upper cervical spinal cord. The hyoid muscles show inspiratory bursts during wakefulness and NREM sleep that are increased by hypercapnia. The relative contribution of hyoid, genioglossus, and other tongue muscles in the maintenance of pharyngeal patency needs to be clarified [162].
Mechanism of Mild Muscle Hypotonia in NREM Sleep
Mild muscle hypotonia in NREM sleep appears to result from a combination of disfacilitation of brain stem motor neurons controlling muscle tone (e.g., mild reduction of activity of locus ceruleus noradrenergic and midline raphe serotonergic neurons) and slight hyperpolarization of brain stem and spinal motor neurons [163]. In addition, there is a direct cerebral cortical mechanism to explain mild muscle hypotonia in NREM sleep, as evidenced by significant enhancement of intracortical inhibition during slow-wave sleep (SWS) after paired-pulse transcranial magnetic brain stimulation [164]. Intracellular microelectrode recording of motor neurons at the onset of NREM sleep by Chase and collaborators [163] clearly showed either no change in membrane potential or a slight hyperpolarization. It should be remembered that the resting membrane potential is determined by an unequal distribution of ions on the outside and the inside of the membrane and by differential permeabilities of the concentration of the sodium, potassium, and chloride ions.
Mechanism of Muscle Atonia or Hypotonia in REM Sleep (See also Chap. 39)
REM sleep is characterized by almost complete cessation of voluntary muscle tone in the presence of a highly active forebrain (paralyzed body with an activated brain) with inhibition of the mesencephalic locomotor region. This is nature’s way of preventing abnormal movements during REM sleep in the presence of highly active cerebral cortex and forebrain regions. The dorsal pontine tegmentum appears to be an important central region responsible for limb muscle atonia in REM sleep [78, 165–167]. Muscle atonia during REM sleep is initiated during activation of a polysynaptic descending pathway from the peri–locus ceruleus alpha in the region of the nucleus pontis oralis to the lateral tegmentoreticular tract, the nucleus gigantocellularis and magnocellularis in the ventral region of the medial medullary reticular formation (the inhibitory region of Magoun and Rhines) [165, 166], and finally, the ventral tegmentoreticular and reticulospinal tracts to the alpha motor neurons causing hyperpolarization and muscle atonia (Fig. 11.3) [167–170]. During REM sleep, an increased number of c-Fos (a nuclear protein synthesized during neuronal activation) labeled cells were detected by immunocytochemical techniques in the inhibitory region of Magoun and Rhines. A key element in the REM sleep-generating mechanism in the pons is the activation of γ-aminobutyric acidergic (GABAergic) neurons located in a subgroup of the pontine reticular formation (sublaterodorsal nucleus [SLD]) as well as GABAergic neurons in the ventrolateral periaqueductal gray region in the mesencephalon [167, 170, 171]. An activation of GABAergic neurons causes excitation or disinhibition of cholinergic neurons, and inhibition of noradrenergic and serotonergic neurons, in the pons. The cholinergic neurons, in turn, excite pontine glutamatergic neurons projecting to the glycinergic premotor neurons in the medullary reticular formation, causing hyperpolarization of the motor neurons and muscle paralysis during REM sleep. This GABAergic mechanism also plays an important role in motor neuron hyperpolarization (see later). Based on more recent study Luppi et al. [171] concluded that REM-on SLD neurons are predominantly glutamatergic. Ventral SLD sends both direct and indirect projections via the ventromedial medulla to brain stem and spinal motoneurons causing hyperpolarization of these neurons using GABA and glycine inhibitory neurotransmitters. During REM sleep, there is also disfacilitation of motor neurons as a result of reduction of the release of midline raphe serotonin and locus ceruleus noradrenaline partially contributing to muscle atonia. Additional contribution to REM atonia comes from lateral hypothalamic hypocretinergic neurons (see further on). Finally, a cerebral cortical mechanism may also contribute to the inhibition of spinal motor neurons in REM sleep, as evidenced by decreased intracortical facilitation in the paired-pulse transcranial magnetic brain stimulation techniques [164].
In summary, there are four fundamental mechanisms responsible for muscle atonia in REM sleep: inhibitory postsynaptic potentials (IPSPs) causing postsynaptic inhibition of motor neurons (major mechanism); disfacilitation (i.e., a reduction of excitation) of presynaptic spinal excitatory neurons; disfacilitation of brain stem and spinal motor neurons controlling muscle tone; and decreased intracortical facilitation (e.g., paired-pulse brain magnetic stimulation technique). During wakefulness and NREM sleep, there are a few spontaneously occurring low-amplitude IPSPs, but during REM sleep, in addition to an increase in these low-amplitude IPSPs, high-amplitude REM sleep-specific IPSPs are noted. These are generated by sleep-specific inhibitory interneurons located mainly in the brain stem (immunocytochemical techniques are used to prove this observation) that send long-projecting axons to the spinal cord and short axons to the brain stem motor neurons [165–170]. As a result of these IPSPs, motor neurons are hyperpolarized by 2–10 mV during REM sleep. Intracellular recordings reveal increased number and appearance of REM sleep-specific IPSPs in the lumbar motor neurons of cats [163, 172–176]. Thus postsynaptic inhibition of motor neurons is responsible for the atonia of somatic muscles, as evidenced by intracellular recordings of spinal motor neurons in chronic spinal preparations of cats. These potentials are derived from inhibitory interneurons, possibly located either in the spinal cord or in the brain stem, from which long axons project to the spinal motor neurons [165, 174, 176]. In addition, there is also postsynaptic inhibition causing a decrease in the Ia monosynaptic excitatory postsynaptic potentials (EPSPs), resulting in motor neuron hyperpolarization. Lesions of the dorsal pontine tegmentum abolish muscle atonia of REM sleep [173–179]. Similar episodes of REM sleep without muscle atonia have also been observed in cats with localized lesions in the ventromedial medulla [180].
Intermittently during REM sleep, there are excitatory drives causing motor neuron depolarization shifts as a result of EPSPs [163, 173–176]. Muscle movements caused by these excitatory drives during REM sleep are somewhat different from the movements noted during wakefulness. These movements are abrupt, jerky, and purposeless. EPSPs during REM sleep reflect increased rates of firing in the motor facilitatory pathways during REM sleep. Enhanced IPSPs during REM sleep check these facilitatory discharges, thus balancing the motor system during this activated brain state; otherwise the blind, unconscious subject will jump out of bed, as may happen in pathologic conditions such as REM sleep behavior disorders [163, 181]. Facilitatory reticulospinal fibers are responsible for transient EPSP phasic discharges causing muscle twitches in REM sleep. Corticospinal or rubrospinal tracts are not responsible for these twitches because Pompeiano showed in 1967 that destruction of these fibers in cats [182] did not affect these twitches.
What neurotransmitters drive these IPSPs? Glycine, a major inhibitory neurotransmitter, was originally thought to be the only driving force. The elegant work by Chase and Morales [163, 183] suggested that glycine is the main neurotransmitter responsible for motor neuron hyperpolarization and IPSPs. The REM sleep-specific IPSPs are reversed after strychnine (a glycine antagonist) administration by microiontophoretic application into the ventral spinal cord [163]. In contrast, picrotoxins and bicuculline (a GABA A antagonist) did not abolish these IPSPs. Recent evidence, however, suggests an important contribution by GABA in addition to glycine [184–189]. Brooks and Peever [188, 189] have shown in their experimental studies in rats that GABA A, GABA B and glycine receptors play a role in mediating REM muscle atonia. It is notable that, in the experiments by Chase and Morales, although the GABA antagonist picrotoxin did not reverse REM sleep-related IPSPs, it reduced the IPSP duration considerably. According to Nitz and Siegel [190, 191], there is a selective GABAergic inhibition of noradrenergic and serotonergic neurons during REM sleep accounting for cessation of discharge of these aminergic cells. As a result of this cessation, there is disfacilitation of motor neurons. GABA may also have a direct inhibitory effect on interneurons and motor neurons. Experimental studies in rats by Besnard et al. [192] and Kubin [193] also provided evidence of wakefulness-related serotonergic and noradrenergic excitatory drive to hypoglossal neurons and withdrawal (disfacilitation during REM sleep).
What is the role of hypocretin in REM motor atonia? Hypocretinergic neurons located in the lateral hypothalamus play a facilitatory role in the motor system by direct projections to the motor neurons and indirectly through projections to the monoaminergic, and cholinergic neurons [163, 194–197]. Hypocretinergic neurons also project to REM generating reticularis pontis oralis nucleus (REM-on) in the Pons and to REM-off neurons in the Pons to suppress REM sleep [198]. Hcrt neurons facilitate motor activity during wakefulness but enhance motor inhibition during REM sleep. There is withdrawal of Hcrt activation of the locus ceruleus noradrenergic and midline raphe serotonergic neurons during REM sleep, as well as withdrawal of Hcrt activation of the spinal motor neurons causing disfacilitation of these brain stem and spinal motor neurons contributing to muscle atonia.
REM Sleep-Related Alterations in Respiratory Muscle Activity
During REM sleep, activity of upper airway muscles and the diaphragm is reduced. Three types of REM sleep-related alterations in the respiratory muscles have been described [199]:
- 1.
- 2.
Rhythmic activity of the diaphragm persists in REM sleep, but certain diaphragmatic motor units cease firing. Kline et al. [201] described intermittent decrement of diaphragmatic activity during single breaths. Upper airway muscles also show similar changes.
- 3.
Fractionations of diaphragmatic activity refer to pauses lasting 40–80 ms and occur in clusters correlated with pontine-geniculate-occipital waves, which are phasic events of REM sleep [202].
What is the mechanism of muscle atonia in the upper airway muscles during REM sleep? Postsynaptic inhibition of motor neurons during REM sleep as described previously is a critical mechanism mediating suppression of hypoglossal motor neurons during REM sleep. Kodama et al. [184], Nitz and Siegel [190, 191] as well as Brooks and Peever [188, 189] suggested that both glycine and GABA play important roles in the regulation of upper airway and postural muscles. A combination of decreased monoamines (e.g., noradrenaline and serotonin) and increased GABA release in the motor neuron pools may be involved in the REM sleep muscle atonia. Fenik et al. [185, 186] as well as several other authors [192, 193, 203–209] suggested that the suppression of upper airway motor tone, including the genioglossus muscle tone, during REM sleep is caused by withdrawal of excitation mediated by norepinephrine and serotonin. Fenik et al. [185, 186] concluded that suppression of motor activity or muscle atonia of the hypoglossus and other upper airway dilator muscles is caused by all or some of the following mechanisms: the withdrawal of motor neuronal excitation mediated by norepinephrine and serotonin, and increased inhibition mediated by GABA and glycine. In summary, the selective inhibition of monoaminergic and orexinergic (hypocretinergic) systems (disfacilitation) coupled with direct active inhibition of motor neurons by GABA and glycine produces a loss of postural muscle tone.
Summary and Clinical Relevance
There is considerable reduction of the activity of the upper airway dilator muscles during NREM sleep, with further reduction in REM sleep, causing increased upper airway resistance and narrowing of the upper airway space. Increased upper airway resistance, which is noted during sleep in normal individuals, may predispose to upper airway occlusion and OSA in susceptible individuals [100]. The site of the upper airway obstruction in OSA is usually at the level of the soft palate, but in approximately half the patients the obstruction extends caudally to the region of the tongue, with further caudal extension during REM sleep [210–218]. Therefore, decreased tone in the palatal, genioglossal, and other upper airway muscles causing increased upper airway resistance and decreased airway space plays an important contributing role in upper airway obstruction in OSA, particularly because many OSA patients have smaller upper airways than individuals without OSA [210, 219–222]. An understanding of these physiological changes in the genioglossus and other upper airway muscles stimulated successful development of hypoglossal nerve stimulation systems for treatment of OSA patients [223]. As a result of the complex effects of sleep on respiration, there is an overall reduction in ventilation during sleep compared to wakefulness [100]. This may not significantly affect a normal person, but may cause life-threatening hypoxemia and abnormal breathing patterns during sleep in patients with neuromuscular disorders, chronic obstructive pulmonary disease, and bronchial asthma, especially in those with daytime hypoxemia [100]. The chemoreflex control of breathing may vary across patients with OSAS. In patients with an apnea-hypopnea index of greater than 30, Mahmed et al. [224]. have shown a significant overnight increase in chemoreflex sensitivity of 30 %, which is another contributing factor toward destabilization of breathing during sleep in this condition. The circadian changes of mild bronchoconstriction during sleep in normal individuals may be accentuated in patients with asthma, causing a marked decrease in peak flow rate, which may in turn cause severe bronchospasm [100, 127].
In summary, during sleep there is a reduction of metabolism and sympathetic activity as a result of which there is depression of respiration, circulation and other vital functions [225]. Following suggested physiological factors may be responsible for breathing dysfunction in sleep [224, 225]:
- 1.
Reduction of skeletal muscle or upper airway muscle tone;
- 2.
Reduced hypoxic and hypercapnic ventilator response;
- 3.
Loss of the wakefulness stimulus causing functional changes in the CNS and reduced respiratory neuronal drive;
- 4.
Fluctuation of excitatory and inhibitory impulses on breathing during various stages of sleep and transition to sleep;
- 5.
Reduced FRC;
- 6.
Ventilation/perfusion (V/Q) mismatch related to body position.
These physiological mechanisms do not affect normal healthy individuals but may worsen breathing in compromised individuals (e.g., those with narrow upper airway space or altered central ventilator stability [i.e., those with high loop gain]).
Physiological Changes in the Heart and Circulation During Sleep
Physiological changes in the heart during sleep include alterations in HR and cardiac output. Changes in circulation during sleep include changes in BP, peripheral vascular resistance (PVR), and blood flow to various systems and regions [226, 227]. All these cardiovascular hemodynamic changes are controlled by the ANS. Briefly, sympathetic inhibition is associated with a decrease in BP and HR during NREM sleep, whereas in REM sleep, intermittent activation of the sympathetic system accounts for rapid fluctuations in BP and HR [38].
Heart Rate
The HR decreases by 5–8 % during NREM sleep and shows frequent upward and downward swings during REM sleep [18, 20, 227–236]. Bradycardia during NREM sleep results from a tonic increase in parasympathetic activity (sympathectomy has little effect) [18, 230, 231]. Bradycardia persists during REM sleep and becomes intense owing to tonically reduced sympathetic discharge. Phasic HR changes (bradytachycardia) during REM sleep are due to transient changes in both the cardiac sympathetic and parasympathetic activities. [18, 228–231]. Thus parasympathetic activity predominates during sleep, and an additional decrease with intermittent increase in sympathetic activity is observed during REM sleep.
In several studies, the HRV during sleep stages has been documented after spectral analysis [20, 231–233, 235]. The documentation of the HF component of the electrocardiogram clearly indicates the prevalence of parasympathetic activity during both NREM and REM sleep. These studies also show intermittent increases of LF components in the electrocardiogram, indicating intermittent sympathetic nervous system activation during REM sleep. Studies also show that the HR acceleration occurs at least 10 beats before EEG arousal [231].
The effect of sleep on cardiac rhythm has been studied in healthy individuals using Holter monitor (see Chap. 47).
Cardiac Output
Cardiac output falls progressively during sleep, with the greatest decrement occurring during the last sleep cycle, particularly during the last REM sleep cycle early in the morning [228, 237]. This may help explain why normal individuals and patients with cardiopulmonary disease are most likely to die during the hours of early morning (see Chap. 47). Maximal oxygen desaturation and periodic breathing are also noted at this time.
Systemic Arterial Blood Pressure
BP falls by approximately 5–14 % during NREM sleep and swings up and down during REM sleep with an overall increase by about 5 % above that noted in NREM sleep. There is a sharp rise in BP around the time of awakening [228, 229, 238–240]. These changes are related to alterations in the ANS [18]. Coote [241] concluded that the fall in BP during NREM sleep was secondary to a reduction in cardiac output, whereas the BP changes during REM sleep resulted from alterations in cardiac output and PVR.
Pulmonary Arterial Pressure
Pulmonary arterial pressure rises slightly during sleep. During wakefulness, the mean value is 18/8 mm Hg; that during sleep is 23/12 mm Hg [242].
Baroreflex Function
There is alteration of baroreflex function, measured indirectly by BRS during sleep as described above.
Peripheral Vascular Resistance
Systemic Blood Flow
Cutaneous, muscular, and mesenteric vascular blood flow shows little change during NREM sleep, but during REM sleep, there is profound vasodilation resulting in increased blood flow in the mesenteric and renal vascular beds [36–39, 228, 244, 245]. However, there is vasoconstriction causing decreased blood flow in the skeletal muscular and cutaneous vascular beds during REM sleep [36–39, 244]. Mullen et al. [246] reported a decrease of plasma renin activity (PRA) in humans during REM sleep, which indirectly suggests increased renal blood flow.
Cerebral Blood Flow
Cerebral blood flow (CBF) and cerebral metabolic rate for glucose and oxygen decrease by 5–23 % during NREM sleep, whereas these values increase to 10 % below up to 41 % above the waking levels during REM sleep [247–255]. These data indirectly suggest [247, 255] that NREM sleep is the state of resting brain with reduced neuronal activity, decreased synaptic transmission, and depressed cerebral metabolism, both glucose and brain oxygen metabolism. CBF is noted to be lower in NREM than in REM sleep, lower at the end of the night compared with that at the beginning of the night, and lower in postsleep wakefulness than presleep wakefulness [255]. Cerebral metabolic rate for oxygen and glucose also decreases in postsleep wakefulness and toward the end of the night compared with the values noted in presleep wakefulness and the beginning of the night [255]. This decreased metabolism, reduced CBF, and reduced anaerobic glycolysis (i.e., there is a greater decrease in glucose utilization compared with oxygen utilization) all support restorative functions of sleep [255].
In the last decade, Maquet and his group (see also Chap. 21) and others[256–274] have made significant contributions using positron emission tomography with [15O]-labeled water or [18F]fluorodeoxyglucose and functional magnetic resonance imaging (MRI) to understand the functional neuroanatomy in normal human sleep. These studies have shown major differences of brain activation during wakefulness, NREM sleep, and REM sleep. During NREM sleep, there is a global decrease in CBF with a regional decrement of CBF in the dorsal pons, mesencephalon, thalami, basal ganglia, basal forebrain, anterior hypothalamus, prefrontal cortex, anterior cingulate cortex, and precuneus [263–265]. These studies confirmed in humans the existence of brain stem–thalamocortical circuits responsible for the NREM sleep-generating mechanisms and correlate with the electrophysiological findings of hyperpolarization of thalamic neurons with generation of sleep spindles, K complexes, and delta and very slow oscillations [260, 265]. The pattern of deactivation in NREM sleep is not homogeneous. The least active areas in NREM sleep were noted in the dorsolateral prefrontal (DLPF) and orbito-frontoparietal and, less consistently, the temporal and insular regions [257, 260, 264]. The primary cortices are the least deactivated areas [264]. However, recent advances in neuroimaging techniques capturing images with higher spatial and temporal resolution have shown that NREM sleep state may not particularly be a state of brain quiescence. Using simultaneous EEG/ event-related functional magnetic resonance imaging (fMRI), Macquet and his group [274, 275] have detected regionally specific increase in activities during SWS in brain stem, cerebellum, ventral prefrontal cortex, posterior cingulate cortex/precuneus and parahippocampal areas in addition to areas generating fast and slow spindles. These findings suggest that during NREM sleep, brain is actively involved in generating synchronized slow waves and spindles which have important implications for memory processing.
In contrast, REM sleep represents an active brain state with increased neuronal activity and increased metabolism. The largest increases during REM sleep are noted in the hypothalamus and the brain stem structures, and the smallest increases are in the cerebral cortex and white matter. REM sleep is characterized by increased neuronal activity, energy requirements, and CBF with regional activations in the pontine tegmentum, thalamus, amygdala, anterior cingulate cortex, hippocampus, temporal and occipital regions, basal forebrain, cerebellum, and caudate nucleus [274]. In contrast, there is regional deactivation in the DLPF, posterior cingulate gyrus, precuneus, and inferior parietal cortex [256, 264, 266]. REM sleep-related activation of the pontine tegmentum, thalamic nuclei, and basal forebrain supports the REM sleep-generating mechanisms in these regions [270]. An activation of limbic and paralimbic structures including the amygdala, hippocampal formation, and anterior cingulate cortex supports the modulatory role of these structures during REM sleep during generation of pontine-geniculate-occipital waves, a major component of phasic REM sleep and HRV in REM sleep [263], and participation of REM sleep in memory processing. REM sleep also showed regional deactivation in the DLPF, precuneus, posterior cingulate cortex, temporoparietal region, and inferior parietal lobule [256, 264, 273, 274].
In the resting state, there are intense neuronal activities both in wakefulness and sleep that can be detected in fMRI as fluctuations of the blood oxygen level-dependent (BOLD) fMRI. These resting state networks (RSN) in sleep and wakefulness showing spontaneous fluctuating neuronal activities suggest that these RSN play an important role in synaptic homeostasis and brain function [276–278].
There are three mechanisms controlling CBF [279]: cerebral autoregulation, cerebral metabolism, and respiratory blood gases (arterial PO2 and arterial PCO2). Cerebral autoregulation is determined by the intrinsic properties of the muscles of the cerebral arterioles. Cerebral autoregulation is normally maintained between the mean systemic arterial pressures of 150 and 60 mm Hg [279]; as the systemic BP falls, cerebral blood vessels dilate in response to changes in transmural pressure, whereas in cases of a rise in BP, the cerebral vessels constrict, thus protecting the brain from fluctuations in systemic BP. This systemic autoregulation may break down in disease states such as stroke, encephalitis, hypertensive crisis, acute head injury, and excessive antihypertensive therapy [279].
There is circadian variation in BP: a reduction during sleep and an increased awakening [280, 281]. Dipping of BP of 10–20 % during sleep compared to mean awake daytime values is physiologic, and these individuals are called “dippers” [280, 281]. There are certain individuals in whom the nocturnal systolic BP during sleep does not fall below 10 % of baseline waking value, and they are known as “nondippers”. There are also individuals known as “reverse dippers” (“risers”) in whom BP does not drop but actually increases during sleep periods. Those individuals considered “extreme dippers,” in whom BP falls excessively, as well as nondippers and reverse dippers, are at higher risk for stroke than dippers [280–283]. Finally, both hypercapnia and hypoxia would cause vasodilation, with hypercapnia causing stronger vasodilation in the cerebral circulation.
Summary and Clinical Implications
These hemodynamic changes in the cardiovascular system result from alterations in the ANS [10, 12, 18, 46, 241]. In general, parasympathetic activity predominates during both NREM and REM sleep and is most predominant during REM sleep. In addition, there is sympathetic inhibition during REM sleep. The sympathetic activity during REM sleep is decreased in cardiac, renal, and splanchnic vessels but increased in skeletal muscles, owing to an alteration in the brain stem sympathetic controlling mechanism. Furthermore, during phasic REM sleep, BP and HR are unstable owing to phasic vagal inhibition and sympathetic activation resulting from changes in brain stem neural activity. HR and BP therefore fluctuate with up and down swing of the BP and HR during REM sleep. Because of these hemodynamic and sympathetic alterations during REM sleep, which is prominent during the last third of total sleep in the early hours of the morning, increased platelet aggregability, plaque rupture, and coronary arterial spasm could be initiated, possibly triggering thrombotic events causing myocardial infarction, ventricular arrhythmias, or even sudden cardiac death [282–285] (see Chaps. 11 and 47). As stated previously, those patients who are nondippers, extreme dippers, and reverse dippers are at higher risk for cardiovascular or cerebrovascular events causing infarctions and periventricular hyperlucencies on MRI. Meta-analysis of epidemiologic studies provides support to the circadian variation in cardiovascular and cerebrovascular events, with the highest rates of events occurring during the early morning hours [284, 285].
Gastrointestinal Physiology During Sleep
A brief summary of the physiology of the gastrointestinal tract during sleep is given in this section. For a more detailed discussion, readers are referred to the writings of Orr [286–289]. Gastrointestinal changes include alterations in gastric acid secretion, gastric volume and motility, swallowing, and esophageal peristalsis and intestinal motility (Table 11.3).
Studying the physiology of the gastrointestinal system has been difficult traditionally because of the lack of adequate technique. Techniques as well as facilities for making simultaneous polysomnographic (PSG) recordings are now available, allowing study of the alterations in gastrointestinal physiology during different stages of sleep. Before the advent of these techniques, scattered reports generally showed decreased motor and secretory functions during sleep. Subsequent methods have produced better and more consistent results, although findings are still somewhat contradictory overall. There is a dearth of adequate studies using PSG and other modern techniques to understand the physiological alterations of gastrointestinal motility and secretions during sleep. The activity of the gastrointestinal tract is controlled by the ANS and the enteric nervous system which is an intrinsic system within the walls of the visceral organs that work closely with the ANS.
Esophageal Function
There are profound alterations in esophageal function during sleep [289–292]. Gastroesophageal reflux (GER) is the most common upper gastrointestinal problem. GER occurs most commonly postprandially during wakefulness, but also occurs during sleep but is less frequent. The availability of the method to measure GER during sleep by 24-h esophageal pH monitoring [293] has advanced our understanding of esophageal function and swallowing during sleep. Waking reflux events are rapidly cleared within 1–2 min, whereas sleep reflux events persist longer, causing longer acid contact. Sleep alters normal response to acid mucosal contact [294]. During wakefulness GER causes increased salivary flow and increased swallowing with the complaints of heartburn. In contrast, during sleep salivary flow and swallowing are considerably decreased, causing prolongation of acid mucosal contact. This predisposes to development of esophagitis, for which there are two other factors: decreased esophageal peristalsis in sleep, particularly SWS, and proximal migration of gastric contents in the distal part of the esophagus [289]. The refluxed acid contents are harmful not only to the esophagus but also to the tracheobronchial tree [286–289, 295].
There are two esophageal sphincters, the upper esophageal sphincter (UES) and the lower esophageal sphincter (LES), acting as barriers to reflux. The third barrier is the epiglottis [295]. The LES is the primary barrier to GER, and both the UES and the LES act as barriers to pharyngoesophageal reflux [289, 295, 296]. In a recent paper, Eastwood et al. [297] simultaneously monitored the functions of the LES and UES during PSG studies in 10 normal volunteers and found a decrement of UES pressure during SWS, particularly in the expiratory phase of breathing. In contrast to other investigators [289, 295, 298], Eastwood et al. [297] did not find an alteration of LES pressure. UES pressure is generated mainly by the cricopharyngeus and to a certain extent by inferior pharyngeal constrictor muscles. LES pressure is generated by contractions of the esophageal smooth muscles and the diaphragm. Pandolfino et al. [298] studied 15 normal subjects using a solid-state high-resolution manometry recording from the hypopharynx to the stomach with simultaneous measurement of lower esophageal pH. These authors noted that the majority of postprandial transient LES relaxations were associated with brief periods of UES relaxations.
The relationship of GER and sleep is reciprocal: sleep affects GER and GER in turn affects sleep. Sleep-related prolonged esophageal acid clearance and acid-mucosa contact result from several sleep-related physiological alterations, which include decreased salivary production and swallowing as well as decreased conscious perception of heartburn and arousal, and delayed gastric emptying [286–289]. In normal individuals who experience episodes of GER, there is generally a reduction in LES pressure [286–289, 295, 296]. Lipan et al. [299] postulated that reflux may advance to the laryngopharynx and into the nasopharynx and paranasal sinuses as a result of a laryngopharyngeal reflux. These authors suggested that breakdown of the following barriers to reflux may cause the laryngopharyngeal reflex: the LES and UES, esophageal motility, esophageal acid clearance, and pharyngeal and laryngeal mucosal resistance. This suggestion is in agreement with that of Orr [295].
Gastric Acid Secretion
During wakefulness, gastric acid secretion depends on food ingestion, increased salivation, and the activity of the gastric vagus nerve. Moore and Englert [300] and Orr [289, 295] showed a clear circadian rhythm for gastric acid secretion in humans. Moore and Englert [300] noted peak gastric acid secretion between 10:00 p.m. and 2:00 a.m. in patients with duodenal ulcer. Figure 11.10 (adapted from Moore and Halberg [301]) schematically shows mean 24-h values for gastric acid secretion in patients with peptic ulcer and normal controls. Acid secretion increases in these patients considerably during the day and at night [302, 303]. The importance of vagal stimulation for the control of circadian oscillation of gastric acid secretion has been demonstrated by the absence of circadian rhythm for gastric acid secretion following vagotomy [304].


Fig. 11.10
Mean 24-h values for gastric acid secretion from patients with active peptic ulcer disease (dark blue rectangles) and normal controls (light blue circles) shown schematically. The ordinate shows hydrochloric acid (Hþ) secretion in milliequivalents (Adapted from Moore and Halberg [301])
Several studies have attempted to understand gastric acid secretion during different stages of sleep, but the results have not been consistent because of methodological flaws and cumbersome techniques [289, 290, 301–308]. An important study was made by Orr et al. [308], who examined five duodenal ulcer patients for five consecutive nights using PSG technique and continuous aspiration of gastric contents. They found no relationship between acid secretion and different stages of sleep or REM versus NREM sleep. The most striking finding was failure of inhibition of acid secretion during the first 2 h of sleep, a result that agrees with the previous study by Levin and associates [302].
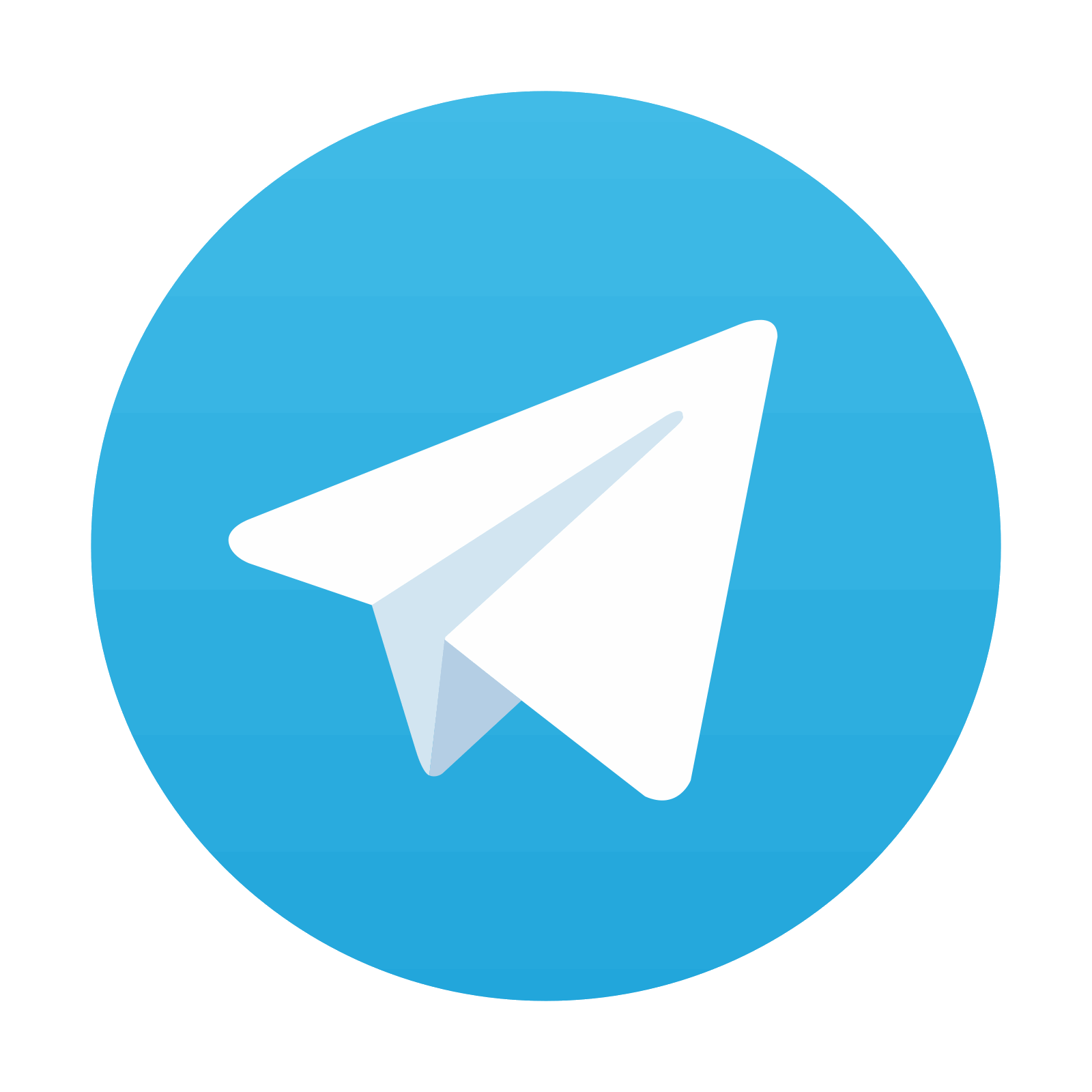
Stay updated, free articles. Join our Telegram channel
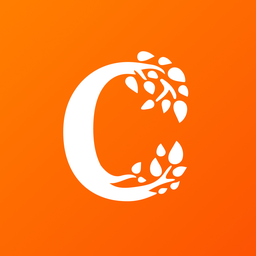
Full access? Get Clinical Tree
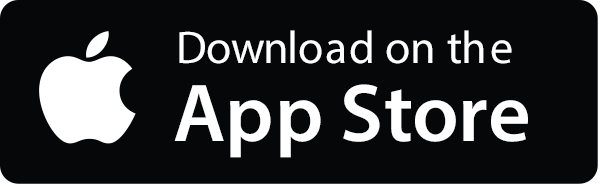
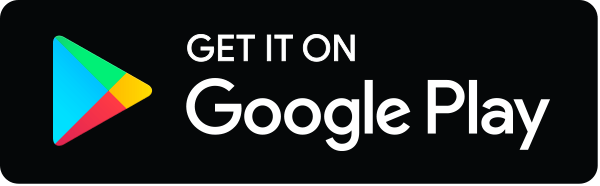