Prefrontal Cortex, Hippocampus, and the Biology of Explicit Memory Storage
Working Memory Depends on Persistent Neural Activity in the Prefrontal Cortex
Intrinsic Membrane Properties Can Generate Persistent Activity
Working Memory Depends on the Modulatory Transmitter Dopamine
Explicit Memory in Mammals Involves Different Forms of Long-Term Potentiation in the Hippocampus
Long-Term Potentiation in the Mossy Fiber Pathway Is Nonassociative
Long-Term Potentiation in the Schaffer Collateral Pathway Is Associative
Long-Term Potentiation in the Schaffer Collateral Pathway Follows Hebbian Learning Rules
Spatial Memory Depends on Long-Term Potentiation in the Hippocampus
A Spatial Map of the External World Is Formed in the Hippocampus
Memory Also Depends on Long-Term Depression of Synaptic Transmission
Are There Molecular Building Blocks for Learning?
EXPLICIT MEMORY—THE CONSCIOUS recall of information about people, places, and objects—is what people commonly think of as memory. Sometimes called declarative memory, it binds our mental life together by allowing us to recall at will what we ate for breakfast, where we ate it, and with whom. It allows us to join what we did today with what we did yesterday or the week or month before that.
The two structures in the mammalian brain that are critical for encoding and storing explicit memories are the prefrontal cortex and the hippocampus. The prefrontal cortex mediates working memory (see Chapter 65). Information stored in working memory can be actively maintained for very short periods and then rapidly forgotten, such as a telephone number that is remembered only until it is dialed, or it can be stored elsewhere in the brain as long-term memory. The hippocampus stores declarative information in a more stable form for periods ranging from days to weeks to years, up to a lifetime. The ultimate storage site for all declarative memories is thought to be in the cerebral cortex. In this chapter we focus on the cellular and molecular mechanisms underlying working memory and long-term storage of explicit memories.
Working Memory Depends on Persistent Neural Activity in the Prefrontal Cortex
In vivo electrophysiological recordings from neurons in the prefrontal cortex of nonhuman primates have provided insights into the neural basis of working memory. Neuronal activity is measured while the animal is engaged in a delayed match-to-sample working memory task. In such tasks the animal is initially shown an image (the sample) and must retain the image in working memory for seconds to minutes after the initial image is extinguished (the delay period). The monkeys are then shown a test image and must press a lever to indicate whether the test image matches the sample image.
Neurons in the prefrontal cortex fire persistently during the delay period, presumably contributing to the neural representation of the image in working memory. Two major mechanisms may contribute to this persistent neural activity: the intrinsic properties of neuronal membranes and recurrent synaptic connectivity.
Intrinsic Membrane Properties Can Generate Persistent Activity
In some cortical neurons a brief electrical stimulation can lead to persistent firing that lasts for seconds or even minutes after the end of the stimulus (Figure 67–1A). Moreover, the rate of firing can be a graded function of the intensity of the stimulation. This persistent firing is not affected by blockers of fast excitatory and inhibitory synaptic transmission, indicating that it depends on the intrinsic membrane properties of the neuron.
Figure 67–1 Mechanisms of persistent neuronal activity that may contribute to working memory. When a monkey performs a working memory task neurons in prefrontal cortex fire persistently during the delay period of the task.
A. Intrinsic mechanisms of graded persistent activity. A brief depolarizing stimulus to a pyramidal neuron in the entorhinal cortex elicits a short burst of action potentials followed by an afterdepolarization (ADP) (1). A slightly longer stimulus elicits a longer burst of spikes followed by a larger afterdepolarization (2). When the stimulus is further lengthened, the afterdepolarization is sufficient to trigger additional action potentials, leading to persistent firing for tens of seconds (3). The diagram illustrates a potential mechanism for the persistent firing. The influx of Ca2+ through voltage-gated Ca2+ channels (VGCC) during an action potential opens Ca2+ -activated nonselective cation (CAN) channels. The resulting inward current through the CAN channels produces an afterdepolarization that can lead to action potentials. The action potentials further activate VGCCs, perpetuating the cycle. The recordings were obtained in the presence of carbachol, which activates muscarinic acetylcholine (ACh) receptors and a downstream signaling cascade that enables the opening of CAN channels when intracellular Ca2+ is also elevated. (Reproduced, with permission, from Egorov et al. 2002.)
B. Recurrent networks of synaptically coupled neurons can lead to persistent reverberatory activity. 1. Some network interactions occur between two widely separated populations of excitatory neurons in distinct brain regions. 2. Other excitatory networks are local, illustrated here by reciprocally connected neighboring pyramidal neurons in neocortex. 3. Persistent activity can be generated through mutual inhibition. This example consists of two populations of neurons, groups 1 and 2. Within each population the neurons are reciprocally connected by excitatory synapses. However, each population mutually inhibits the other. In this manner an excitatory input to group 1 leads to the silencing of group 2. The loss of inhibitory input from group 2 (a process termed disinhibition) in turn enhances the firing of group 1 (B1 and B2 reproduced, with permission, from Wang 2001; B3 reproduced, with permission, from Aksay et al. 2007.)
The intrinsic mechanism underlying persistent firing has been best characterized in neurons in the deep layers of the entorhinal cortex. Normally a brief depolarizing current pulse elicits a transient burst of action potentials in these neurons. However, when the entorhinal neurons are exposed to acetylcholine, which activates G-protein coupled muscarinic receptors, a brief depolarizing current elicits a prolonged train of action potentials that persists for tens of seconds, far longer than the current stimulus.
This maintained firing depends on the opening of a type of channel termed the Ca2+-activated non-selective (CAN) cation channel. The opening of these channels requires two simultaneous events. First, the muscarinic receptor signaling cascade must be stimulated by extracellular acetylcholine; second, there must be an increase in intracellular Ca2+, normally generated by the opening of voltage-gated Ca2+ channels during the firing of a brief burst of action potentials. Ca2+ then opens the CAN channel by binding to a site on the channel’s cytoplasmic surface. As the cytoplasmic Ca2+ level remains elevated for some time after the burst of action potentials, the inward current through the CAN channels leads to a prolonged afterdepolarization following the burst of action potentials.
If the initial stimulation period is sufficiently intense, the Ca2+ influx will activate sufficient current through the CAN channels so that the afterdepolarization will trigger a second round of spikes. This in turn leads to more Ca2+ influx, which activates more CAN channels, leading to a larger afterdepolarization that can maintain firing that far outlasts the initial stimulus. Thus these CAN channels contribute to persistent firing by participating in a positive feedback loop with voltage-gated Ca2+ channels. Recent studies suggest that this mechanism of persistent firing also is observed in prefrontal cortex neurons.
Network Connections Can Sustain Activity
The second type of mechanism for sustained firing depends on recurrent synaptic connections within neural circuits. In the simplest case activity is maintained by recurrent excitatory connections within the active population of neurons. A network can comprise either long-range connections between distinct regions of the brain or local circuits (Figure 67–1B). The firing maintained through such chains is referred to as reverberatory activity.
Another circuit that can sustain activity depends on reciprocal inhibitory synapses between two populations of neurons (Figure 67–1B). Neurons in both populations fire spontaneously at a basal level that is normally held in check by the reciprocal inhibitory synapses. However, a brief excitatory input to one population of neurons will transiently enhance their firing rate, which leads to an increase in their inhibitory output onto the second population. As a result, the firing rate of the second population decreases. This decreases the inhibitory input onto the first population of neurons, further enhancing their rate of firing.
This mechanism of positive feedback, termed disinhibition, can lead to firing of the first population of neurons that outlasts the initial stimulus. A network of reciprocal inhibitory connections between distinct populations of neurons contributes to the sustained firing of oculomotor neurons such as those of the goldfish, which are responsible for remembering eye position. It is likely that persistent activity during working memory involves a combination of network and intrinsic mechanisms.
Working Memory Depends on the Modulatory Transmitter Dopamine
Although the relative importance of intrinsic activity versus network activity in working memory remains uncertain, it is clear that the efficiency of working memory and persistent activity in prefrontal cortex neurons depends on the state of activation of the D1 type of dopamine receptors. These receptors are coupled to the G protein Gs and the production of cyclic adenosine monophosphate (cAMP).
Patricia Goldman-Rakic and colleagues have found that there is an inverted U-shaped relation between the extent of D1 receptor activation and working memory: Working memory is most efficacious at intermediate levels of D1 receptor activation. Defects in the dopaminergic regulation of working memory in prefrontal cortex are thought to contribute to the cognitive deficits associated with schizophrenia.
Explicit Memory in Mammals Involves Different Forms of Long-Term Potentiation in the Hippocampus
What neural mechanisms are responsible for long-term explicit memory mediated by the hippocampus and its associated structures in the medial temporal lobe of the mammalian brain? Unlike working memory, long-term storage of information by the hippocampus is not thought to depend on persistent neural firing but rather to involve long-lasting changes in the strength of synaptic connections.
The hippocampus receives multimodal sensory and spatial information from the nearby entorhinal cortex. The major output of the hippocampus is through the pyramidal neurons in the CA1 region, which project back to the entorhinal cortex and to the subiculum, another medial temporal lobe structure. The critical importance of CA1 neurons in learning and memory is seen in the profound memory loss exhibited by patients with lesions in this region, which has been complemented by numerous studies in animal models. Information from the entorhinal cortex reaches CA1 neurons along two excitatory pathways, one direct pathway and one indirect. Together these inputs are termed the perforant pathways.
The direct pathway has its origins in neurons of layer III of the entorhinal cortex. The axons of these neurons form synapses on the very distal apical dendrites of CA1 neurons (such perforant projections are also called the temporoammonic pathway). In the indirect pathway information from neurons of layer II of the entorhinal cortex reaches CA1 neurons through the tri-synaptic pathway. In the initial leg of this pathway the axons of layer II neurons project through the perforant pathway to the granule cells of the dentate gyrus (an area considered part of the hippocampus). The granule cell axons project in the mossy fiber pathway to excite the pyramidal cells in the CA3 region of the hippocampus. Finally, the CA3 axons project through the Schaffer collateral pathway to make excitatory synapses on more proximal regions of CA1 pyramidal cell dendrites (Figure 67–2).
Figure 67–2 The hippocampal synaptic circuit is important for declarative memory. Information arrives in the hippocampus from entorhinal cortex through the perforant pathways, which provide both direct and indirect input to CA1 pyramidal neurons, the major output neurons of the hippocampus. (Arrows denote the direction of impulse flow.) In the indirect trisynaptic pathway neurons in layer II of entorhinal cortex send their axons through the perforant path to make excitatory synapses onto the granule cells of the dentate gyrus. The granule cells project through the mossy fiber pathway and make excitatory synapses with the pyramidal cells in area CA3 of the hippocampus. The CA3 cells excite the pyramidal cells in CA1 by means of the Schaffer collateral pathway. In the direct pathway neurons in layer III of entorhinal cortex project through the perforant path to make excitatory synapses on the distal dendrites of CA1 pyramidal neurons without intervening synapses.
The fact that CA1 pyramidal neurons receive cortical information through two pathways has led to the view that CA1 neurons compare information in the indirect circuit with sensory input from the direct pathway. Lesion studies indicate that both direct and indirect inputs to CA1 may be necessary for normal learning and memory. Lesions of the indirect Schaffer collateral pathway limit the ability of mice to perform a complex spatial learning and memory task, although some form of spatial learning remains intact. Lesions of the direct pathway to CA1 do not appear to alter initial formation of memory, but inhibit the ability of an animal to store those initial memories as long-term memory, a process termed consolidation. Genetic inactivation of the direct path also interferes with episodic memory, in which an animal must learn about the temporal relation between two or more events.
In 1973 Timothy Bliss and Terje LomØ discovered that the initial stage of the trisynaptic pathway—the perforant pathway from layer II of the entorhinal cortex to the dentate granule neurons—is remarkably sensitive to previous activity. A brief high-frequency train of stimuli (a tetanus) gives rise to long-term potentiation (LTP), a long-lasting increase in the amplitude of the excitatory postsynaptic potentials (EPSPs) in the dentate granule neurons. (In Chapter 66 we saw how a similar form of synaptic potentiation at synapses in the amygdala contributes to fear conditioning.) Subsequent studies showed that brief high-frequency trains of stimulation can induce forms of LTP at all three synapses of the trisynaptic pathway as well as at the direct perforant path synapses with CA1 neurons (Figure 67–3). Long-term potentiation can last for days or even weeks when induced in the intact animal using implanted electrodes. LTP can also be examined in slices of hippocampus and in cell culture, where it can last several hours.
Figure 67–3 Different neural mechanisms underlie long-term potentiation at each of the three synapses in the trisynaptic pathway in the hippocampus. Long-term potentiation (LTP) is present at synapses throughout the hippocampus but depends to differing degrees on activation of NMDA-type glutamate receptors.
A. Tetanic stimulation of the Schaffer collateral pathway (at arrow) induces LTP at the synapses between presynaptic terminals of CA3 pyramidal neurons and their postsynaptic CA1 pyramidal neurons. The graph plots the size of the extracellular field EPSP (fEPSP) expressed as a percent of the initial baseline fEPSP prior to induction of LTP. At these synapses LTP requires activation of the NMDA receptors in the CA1 neurons as it is completely blocked when the tetanus is delivered in the presence of the NMDA receptor antagonist APV. (Reproduced, with permission, from Morgan and Teyler 2001.)
B. Tetanic stimulation of the direct pathway from entorhinal cortex to CA1 neurons generates LTP of the fEPSP that depends partially on activation of the NMDA receptors and partially on activation of L-type voltage-gated Ca2+ channels. It is therefore only partially blocked by APV. Addition of APV and nitrendipine, a dihydropyridine that blocks L-type channels, is needed to fully inhibit LTP. (Reproduced, with permission, from Remondes and Schuman 2003.)
C. Tetanic stimulation of the mossy fiber pathway induces LTP at the synapses with the pyramidal cells in the CA3 region. In this experiment the excitatory postsynaptic current was measured under voltage-clamp conditions. This LTP does not require activation of the NMDA receptors and so is not blocked by APV. It does require activation of protein kinase A and so is blocked by the kinase inhibitor H-89. (Reproduced, with permission, from Zalutsky and Nicoll 1990.)
Studies in these different pathways have shown that LTP is not a single form of synaptic plasticity. Rather it comprises a family of processes that strengthen synaptic transmission at different hippocampal synapses through distinct cellular and molecular mechanisms. Indeed, even at a single synapse different forms of LTP can be induced by different patterns of synaptic activity. However, these distinct processes also share many important similarities.
All forms of LTP are induced by synaptic activity in the pathway that is being potentiated—that is, LTP is homosynaptic. However, the various forms of LTP differ in the relative importance of different receptors and ion channels. In addition, different forms of LTP may recruit different second-messenger signaling pathways either in the presynaptic cell, altering transmitter release, or in the postsynaptic cell, altering its sensitivity to the neurotransmitter glutamate.
The similarities and differences in the mechanisms of LTP at the Schaffer collateral, mossy fiber, and entorhinal inputs to CA1 can be seen by examining the role of the postsynaptic NMDA type of glutamate receptor in the induction of LTP in the three pathways. In all three pathways synaptic transmission is persistently enhanced in response to a brief tetanic stimulation. However, the contribution of the NMDA receptor to the induction of LTP differs in the three pathways.
At the Schaffer collateral synapses with CA1 pyramidal neurons, the induction of LTP in response to a brief 100 Hz stimulation is completely blocked when the tetanus is applied in the presence of the NMDA receptor antagonist 2-amino-5-phosphonovaleric acid, (AP5 or APV). However, APV only partially inhibits the induction of LTP at the direct entorhinal synapses with CA1 neurons and has no effect on LTP at the mossy fiber synapses with CA3 pyramidal neurons (Figure 67–3). In the next two sections we consider the mechanisms of LTP in more detail, first in the mossy fiber pathway and then in the Schaffer collateral pathway.
Long-Term Potentiation in the Mossy Fiber Pathway Is Nonassociative
Glutamate released at the mossy fiber synapses binds to both the NMDA and AMPA type of glutamate receptors in the postsynaptic membrane of the CA3 neurons. However, under most conditions the NMDA receptors have only a minor role in synaptic transmission in this pathway. Moreover, as noted above, blocking these receptors has no effect on LTP (Figure 67–3C). Rather, LTP in the mossy fiber pathway is triggered by the large Ca2+ influx into the presynaptic terminals during a tetanus. In the presynaptic cell the Ca2+ influx activates a calcium/calmodulin–dependent adenylyl cyclase complex, thereby increasing the production of cAMP and activating protein kinase A. This leads to an increase in the release of glutamate from the mossy fiber terminals, resulting in LTP. Activity in the postsynaptic cell is not required for this form of LTP. Thus, mossy fiber LTP is nonassociative.
The increase in transmitter release is thought to depend on the ability of protein kinase A to phosphorylate RIM1α, a synaptic vesicle protein that interacts with several other presynaptic proteins important for exocytosis (see Chapter 12). Thus mossy fiber LTP is abolished in mice in which the gene for RIMα, has been deleted through genetic engineering. The importance of presynaptic protein kinase A in mossy fiber LTP resembles aspects of the synaptic changes responsible for associative learning in the gill-withdrawal reflex of Aplysia and amygdala-based learned fear in rodents (see Chapter 66). Another similarity with the synaptic changes in Aplysia is that induction of mossy fiber LTP is under the control of a system of modulatory inputs. Just as the activation of adenylyl cyclase by serotonin is important for long-term facilitation in Aplysia, mossy fiber LTP is facilitated by the binding of norepinephrine to β-adrenergic receptors, enhancing the activation of adenylyl cyclase.
Long-Term Potentiation in the Schaffer Collateral Pathway Is Associative
Like the mossy fiber terminals in the CA3 region, glutamate released from the Schaffer collateral terminals activates both AMPA and NMDA receptors in the postsynaptic membrane of CA1 pyramidal neurons. However, unlike the mossy fiber system, LTP in the Schaffer collateral pathway requires activation of the NMDA receptors in the postsynaptic cell, which triggers a complex postsynaptic signaling cascade.
The opening of the NMDA receptors, unlike the AMPA receptors, requires that two events occur simultaneously. First, like any ionotropic receptor, glutamate must bind to the NMDA receptor to open the channel. However, when the membrane is at the resting potential or only modestly depolarized by a weak synaptic input, glutamate binding by itself is not sufficient for the NMDA receptors to conduct ions because the pore of the receptor-channel is blocked by extracellular Mg2+ (Figure 67–4A; see Chapter 10). For the receptor to function efficiently, the postsynaptic membrane must undergo a significant depolarization to expel the bound Mg2+ by electrostatic repulsion. In this manner the receptor acts as a coincidence detector: It is functional only when action potentials in the presynaptic neuron release glutamate that binds to the receptor and the membrane potential of the postsynaptic cell is sufficiently depolarized.
Figure 67–4 (Opposite) A model for the induction of long-term potentiation at Schaffer collateral synapses.
A. During normal, low-frequency synaptic transmission glutamate released from the terminals of CA3 Schaffer collateral axons acts on both NMDA and AMPA receptors in the postsynaptic membrane of dendritic spines (the site of excitatory input) of CA1 neurons. Sodium and K+ flow through the AMPA receptors but not through the NMDA receptors because their pore is blocked by Mg2+ at negative membrane potentials.
B. During a high-frequency tetanus the large depolarization of the postsynaptic membrane (caused by strong activation of the AMPA receptors) relieves the Mg2+ blockade of the NMDA receptors, allowing Ca2+, Na+, and K+ to flow through these channels. The resulting increase of Ca2+ in the dendritic spine triggers calcium-dependent kinases—calcium/calmodulin– dependent kinase (CaMKII) and protein kinase C (PKC)—as well as the tyrosine kinase Fyn, leading to induction of LTP.
C. Second-messenger cascades activated during induction of LTP have two main effects on synaptic transmission. Phosphorylation through activation of protein kinases, including PKC, enhances current through the AMPA receptors, in part by causing insertion of new receptors into the spine synapses. In addition, the postsynaptic cell releases (in ways that are still not understood) retrograde messengers that activate protein kinases in the presynaptic terminal to enhance subsequent transmitter release. One such retrograde messenger may be nitric oxide (NO), produced by the enzyme NO synthase (shown in part B).
Because of the Mg2+ blockade of the NMDA receptors, at negative voltages near the resting potential EPSPs are largely generated by the opening of AMPA receptors. The burst of strong synaptic activity during induction of LTP opens a large number of AMPA receptors, generating an EPSP that is sufficient to trigger a postsynaptic action potential. The action potential generates a large depolarization that is able to expel Mg2+ from the pore of the NMDA receptor, permitting the receptor to conduct cations and contribute to the postsynaptic depolarization.
Why are the NMDA receptors required to induce LTP if the AMPA receptors are sufficient to produce a large postsynaptic depolarization? The answer lies in the fact that in addition to conducting monovalent Na+, and K+ ions, similar to the conductance properties of the AMPA receptors, the NMDA receptors also have a high permeability to Ca2+. Thus activation of these receptors leads to a significant increase in the intracellular Ca2+ concentration in the postsynaptic cell. The Ca2+ elevation is vital to the induction of LTP; injection of a chemical chelator of Ca2+ into the postsynaptic CA1 cell blocks the induction of LTP. The increase in Ca2+ activates several downstream signaling pathways, including calcium/calmodulin–dependent protein kinase II (CaMKII), protein kinase C (PKC), and tyrosine kinases. These signaling pathways lead to changes that both enhance the response of the postsynaptic cell to glutamate and increase the amount of glutamate released from the presynaptic Schaffer collateral terminals (Figure 67–4B).
Neuroscientists often find it useful to distinguish between the mechanisms underlying the induction of LTP (the biochemical reactions activated by the tetanic stimulation) and those responsible for the expression of LTP (the long-term changes that take place at the synapse responsible for enhanced synaptic transmission). The mechanisms for the induction of LTP at the CA3-CA1 synapse are postsynaptic. What are the mechanisms involved in the expression of LTP at this synapse? Is the enhancement caused by an increase in transmitter release, an increased postsynaptic response to a fixed amount of transmitter, or some combination of the two?
Recent studies suggest that the cellular mechanisms underlying the expression of LTP vary depending on the precise pattern of activity that induces LTP. In many cases LTP that is induced solely by Ca2+ influx through NMDA receptors appears to be largely caused by an increase in the response of the postsynaptic membrane of the CA1 neuron to glutamate. But other patterns of stimulation elicit other forms of LTP at the same synapse and these also have presynaptic effects that enhance transmitter release.
One of the key pieces of evidence for a postsynaptic contribution to the expression of LTP at Schaffer collateral synapses comes from an examination of so-called “silent synapses” (Figure 67–5). In some recordings from pairs of hippocampal pyramidal neurons, stimulation of an action potential in one neuron fails to elicit a synaptic response in a second (postsynaptic) neuron when that neuron is at its resting potential (approximately –70 mV).
Figure 67–5 Unsilencing of silent synapses during long-term potentiation.
A. Intracellular recordings are obtained from a pair of hippocampal pyramidal neurons. An action potential is triggered in neuron a by a depolarizing current pulse and the resultant excitatory postsynaptic current (EPSC) produced in neuron b is recorded under voltage clamp conditions.
B. Effect of induction of LTP on silent synapses. Before induction of LTP there is no EPSC in cell b in response to an action potential in cell a when the membrane potential of neuron b is at its resting value of −65 mV (1). However, slow NMDA receptor-mediated EPSCs are observed when neuron b is depolarized by the voltage clamp to +30 mV (2). LTP is then induced by pairing action potentials in neuron a with postsynaptic depolarization in neuron b to relieve Mg2+ block of the NMDA receptors. After this pairing fast AMPA receptor-mediated EPSCs are seen at −65 mV (3).
C. Mechanism of the unsilencing of silent synapses. Prior to LTP the dendritic spine contacted by a presynaptic CA3 neuron contains only NMDA receptors. Following induction of LTP intracellular vesicles containing AMPA receptors fuse with the plasma membrane at the synapse, adding new receptors on the spine.
This result is not surprising as any given hippocampal presynaptic neuron is connected to only a small fraction of other neurons. However, what is surprising is that, in some neuronal pairs, when the second neuron is depolarized under voltage clamp to +30 mV, which removes the Mg2+ block from the NMDA receptors, stimulation of the presynaptic neuron elicits a large excitatory postsynaptic current (EPSC) in the postsynaptic neuron, mediated by the NMDA receptors. This result indicates that the two neurons were synaptically connected all along but the postsynaptic neuron contained only NMDA receptors at its synaptic contact with the presynaptic neuron. These connections are called silent synapses because they do not generate an EPSP at the normal resting potential of the cell as a result of the Mg2+ block of the NMDA receptors. Synapses from other presynaptic neurons on the same postsynaptic cell may have AMPA receptors in addition to NMDA receptors (nonsilent synapses).
The key finding from these experiments is seen following the induction of LTP. Pairs of neurons initially connected solely by silent synapses now often exhibit large EPSPs at the resting potential mediated by AMPA receptors. These results indicate that LTP must involve an increase in the response of AMPA receptors to glutamate at the previously silent synapses, a process Roberto Malinow refers to as “AMPAfication.”
How does the induction of LTP increase the response of AMPA receptors at previously silent synapses? The strong synaptic stimulation used to induce LTP will trigger glutamate release at both silent and nonsilent synapses on the same postsynaptic neuron. This leads to the opening of a large number of AMPA receptors at the nonsilent synapses, which in turn produces a large postsynaptic depolarization. The depolarization will propagate throughout the neuron to relieve Mg2+ block of the NMDA receptors at both the nonsilent and silent synapses. At the silent synapses the Ca2+ influx through the NMDA receptors activates a biochemical cascade that ultimately leads to the insertion of clusters of AMPA receptors in the postsynaptic membrane from a pool of intracellular receptors stored in recycling endosomal vesicles. The fusion of these vesicles with the plasma membrane is triggered by the phosphorylation by protein kinase C of the cytoplasmic tail of the endosomal AMPA receptors (Figure 67–4B,C).
As discussed earlier, LTP is not a unitary process even at a single synapse. At Schaffer collateral synapses LTP generated by a brief 100 Hz tetanus depends solely on Ca2+ influx through NMDA receptors, whereas LTP induced by a 200 Hz tetanus depends on Ca2+ influx through both NMDA receptors and L-type voltage-gated Ca2+ channels. (A similar mechanism contributes to LTP in the direct entorhinal pathway to CA1 neurons.) This high-frequency form of LTP is expressed both through presynaptic mechanisms that enhance glutamate release and through postsynaptic mechanisms that increase the membrane response to glutamate. Thus both the induction and expression of LTP depend on a family of presynaptic and postsynaptic processes.
Because induction of LTP requires Ca2+ influx into the postsynaptic cell, the increase in transmitter release during LTP implies that the presynaptic cell must receive information from the postsynaptic cell that LTP has been induced. There is now evidence that Ca2+-activated second messengers in the postsynaptic cell, or perhaps Ca2+ itself, cause the postsynaptic cell to release one or more chemical messengers that diffuse to the presynaptic terminals to enhance release (see Figure 67–4B,C and Chapter 11). Importantly, these diffusible retrograde signals appear to affect only those presynaptic terminals that have been activated by the tetanic stimulation, thereby preserving synapse specificity.
Long-Term Potentiation in the Schaffer Collateral Pathway Follows Hebbian Learning Rules
The NMDA receptors endow LTP in the Schaffer collateral pathway with several interesting properties that have direct relevance to learning and memory. First, LTP in this pathway requires the near simultaneous activation of a large number of afferent axons, a feature called cooperativity (Figure 67–6). This requirement stems from the fact that relief of Mg2+ block of the NMDA receptor requires a large depolarization.
Figure 67–6 Long-term potentiation in CA1 pyramidal neurons of the hippocampus shows cooperativity, associativity, and synapse specificity. With normal synaptic transmission a single action potential in one or a few axons (weak input) leads to a small EPSP that is insufficient to expel Mg2+ from the NMDA glutamate receptor-channels and thus cannot induce LTP. This ensures that irrelevant stimuli are not remembered. The near-simultaneous activation of several weak inputs during strong activation (cooperativity) produces a suprathreshold EPSP that triggers action potential firing and results in LTP in all pathways. Stimulation of strong and weak inputs together (associativity) causes LTP in both pathways. In this way a weak input becomes significant when paired with a powerful one. An unstimulated synapse does not undergo LTP in spite of the strong stimulation of neighboring synapses. This ensures that memories are selectively formed at active synapses (synapse specificity).
The second important property of LTP in the Schaffer collateral pathway is that it is associative. A weak synaptic input normally does not produce enough postsynaptic depolarization to induce LTP. However, if that weak input is coactivated or paired with a strong synaptic input that does produce supra-threshold depolarization, then the large depolarization will be able to propagate to the synapse with weak input, leading to relief of the Mg2+ blockade of the NMDA receptors in the postsynaptic membrane at that site and the induction of LTP.
The third key property of LTP is that it is synapse specific. If a particular synapse is not activated during a period of strong synaptic stimulation, the NMDA receptors at that site will not be able to bind glutamate and thus will not be activated despite the strong postsynaptic depolarization. As a result, that synapse will not undergo LTP.
Each of these three properties of cooperativity, associativity, and synapse specificity underlies key components of memory storage. Cooperativity ensures that only events of a high degree of significance, those that activate sufficient inputs, will result in memory storage. Associativity, like associative Pavlovian conditioning, allows an event (or conditioned stimulus) that has little significance in and of itself to be endowed with a higher degree of meaning if that event occurs just before or simultaneously with another more significant event (an unconditioned stimulus). Finally, synapse specificity ensures that inputs that convey information not related to a particular event will not be strengthened to participate in a given memory.
The finding that the induction of LTP in the Schaffer collateral pathway requires that presynaptic activity be strong enough to elicit firing in the postsynaptic neuron provides evidence for Hebb’s rule, proposed in 1949 by the psychologist Donald Hebb as a theoretical mechanism for how neuronal circuits are modified by experience: “When an axon of cell A … excites cell B and repeatedly or persistently takes part in firing it, some growth process or metabolic change takes place in one or both cells so that A’s efficiency as one of the cells firing B is increased.” A similar principle is involved in fine-tuning synaptic connections during the late stages of development (see Chapter 56).
The Hebbian nature of LTP is best illustrated by the phenomenon of spike timing-dependent plasticity. Under most circumstances hippocampal neurons do not produce the high-frequency trains of action potentials typically used to induce LTP. However, a form of LTP can be induced by pairing a single presynaptic stimulus with the firing of a single action potential in the postsynaptic cell. In fact, this coincidence detection is very strict. In agreement with Hebb’s postulate, the pairing protocol produces LTP only if the postsynaptic cell fires a few milliseconds after the EPSP. That is, the presynaptic cell must fire before the postsynaptic cell. If the postsynaptic cell fires just before the EPSP, a long-lasting decrease in the size of the EPSP occurs (this long-term depression is described more fully below.) If the action potential occurs more than a hundred milliseconds before or after the EPSP, the synaptic strength will not change.
The pairing rules of spike timing-dependent plasticity result in large part from the cooperative properties of the NMDA receptor. If the postsynaptic spike occurs during the EPSP, the spike is able to relieve the Mg2+ blockade of the receptor at a time when the NMDA receptor-channel has been activated by the binding of glutamate. This leads to a large influx of Ca2+ through the receptor and the induction of LTP. However, if the postsynaptic action potential occurs prior to glutamate release, any relief from the Mg2+ block will occur when the gate of the receptor-channel is closed, because of the absence of glutamate. As a result there will be little influx of Ca2+ through the receptor to induce LTP.
These studies of the Schaffer collateral pathway indicate that two sequential associative mechanisms ensure that the induction of LTP is restricted to those synapses at which there is both presynaptic and postsynaptic activity, in accord with Hebb’s learning rule. The first mechanism is the associative property of the NMDA glutamate receptor. The second is the selective action of retrograde messengers released from the postsynaptic cell at only those presynaptic sites that are active. As we saw in Chapter 66, these two associative mechanisms in series also contribute to associative classical conditioning in Aplysia and in the amygdala. Thus mechanisms of synaptic plasticity important for learning and memory have been conserved throughout evolution of the species at broad classes of synapses and for distinct forms of learning.
Long-Term Potentiation Has Early and Late Phases
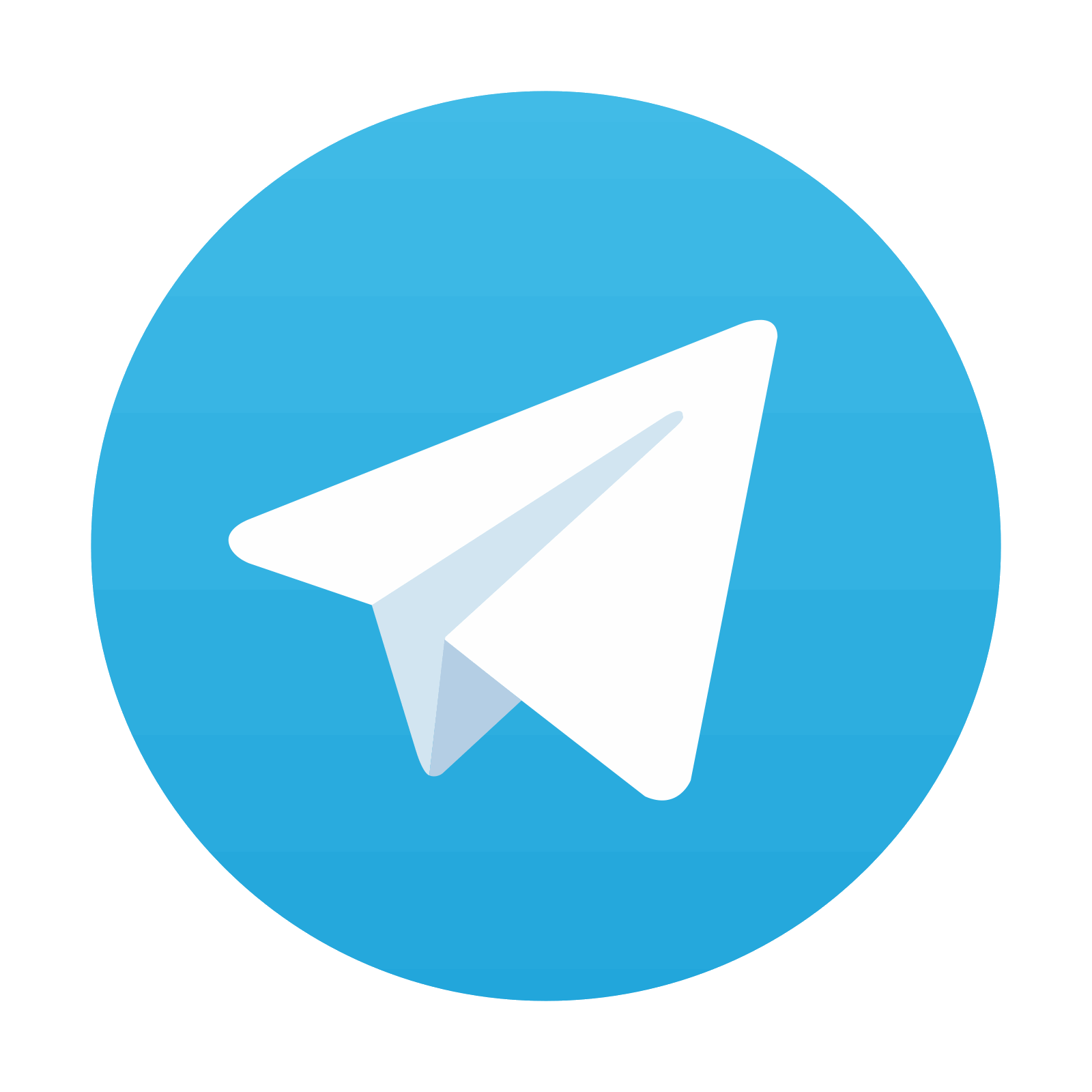
Stay updated, free articles. Join our Telegram channel
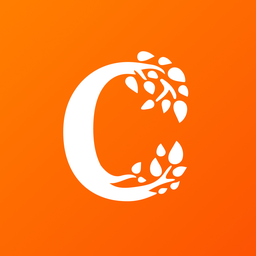
Full access? Get Clinical Tree
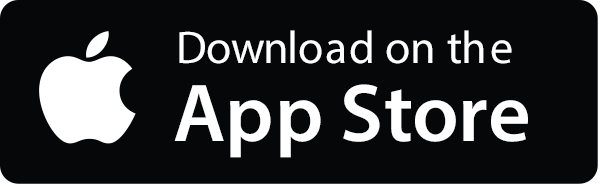
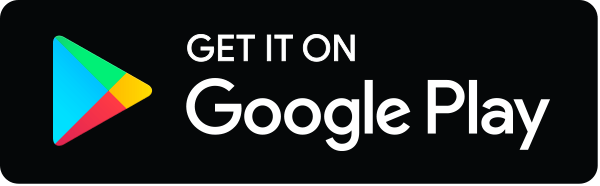