Principles of Pediatric Spinal Column Trauma
The unique anatomical characteristics of the developing spinal column change dramatically from infancy through childhood and into adolescence. These anatomical characteristics directly influence spinal biomechanics, which subsequently influence the response of the pediatric spine to physiologic and pathologic forces. Although prevention is the most effective means to combat pediatric spinal column injuries, a thorough understanding of these relationships is necessary to provide optimal care to children following vertebral column trauma.
This chapter addresses the underlying principles of pediatric spinal column trauma without focusing on specific injury types or the neurologic outcomes of injury. Specifically, the epidemiology, developmental anatomy, biomechanics, initial management, and diagnostic imaging pertaining to traumatic injuries of the immature spinal column are discussed. Additionally, information on the general principles of external and internal immobilization in the treatment of these injuries is included. Specific types of traumatic injury affecting the pediatric spinal column—including injury description, clinical presentation, radiographic diagnosis, and appropriate treatment—are discussed at length in the next chapter.
60.1 Epidemiology
The epidemiology of pediatric spinal column trauma is influenced heavily by patient age, which is closely associated with the incidence of injury, etiology and mechanism of injury, male-to-female ratio of injured patients, the vertebral level most likely to be injured, and the injury pattern.
60.1.1 Frequency
Occurring less frequently than in adults, pediatric spine injuries account for only 5 to 7% of all spine injuries that present to major medical centers.1 The incidence of spine injury in children less than 19 years of age in the United States is approximately 20 injuries per million per year.1 There is a seasonal variation in the incidence of pediatric spinal column trauma in North America, with a peak in the summer months from June to September and an additional increase in incidence during the winter holidays at the end of December and beginning of January.2
60.1.2 Etiology
The etiology of traumatic injuries to the pediatric spinal column varies depending on the age of the patient. Overall, in North America, motor vehicle accidents comprise the single most common cause of injury. In younger children (birth to 9 years of age), falls and pedestrian-versus-vehicle accidents account for as many as 75% of injuries.1,3,4 In children 10 to 14 years of age, motor vehicle accidents become more prevalent and account for 40% of cases, with falls and pedestrian accidents decreasing in frequency. In late adolescence (ages 15 to 17 years), motor vehicle accidents predominate as the leading cause of spinal column injury, accounting for more than 70% of cases in some series. Sports-related injuries also become more frequent, and sports are the second most common etiology in this age group.1,3–6
60.1.3 Gender
The male-to-female ratio of children with spinal column injuries varies with age, in concordance with the shift in etiology. In the youngest age group (birth to 9 years of age), the male-to-female ratio (1.1 to 1.3:1) is the smallest because pedestrian accidents tend to affect both genders equally, whereas boys are slightly more susceptible to falls.1,3,6 The ratio becomes significantly higher as one approaches the 15- to 17-year-old group (2.3 to 2.5:1), presumably because of a propensity of males to participate more frequently in aggressive sports and reckless driving.1,3,6
60.1.4 Level of Injury
The vertebral level of injury in pediatric patients with spinal column trauma is strongly correlated with age, as predicted by the biomechanics of the immature spine. Numerous studies have demonstrated that the majority of spinal cord and vertebral column injuries occur in the cervical region within this age group.7 Two large series of pediatric spinal column injuries reported that the injuries were located in the cervical spine in almost two-thirds of patients (61%), followed by lumbar (14%), thoracolumbar (14%), and thoracic locations (11%).3,7 In particular, younger children are more likely to have upper cervical spine injuries, and as patient age increases, the most frequently injured level moves progressively caudal. Upper cervical and craniovertebral junction (CVJ) injuries are two to three times as frequent in children less than 3 years old than in older children and adults.3,6,8 In contrast to upper cervical injuries, whose frequency is largely influenced by age, lower cervical and thoracic injuries occur with approximately equal frequencies in both younger (birth to 9 years) and older (10 to 17 years) children because maturation of the spine at these regions occurs much more gradually than at the CVJ.6,9 Thoracolumbar and lumbar injuries are primarily lesions of adolescence. Of important consideration in pediatric spinal column trauma is the high incidence of multilevel contiguous (26 to 34%) and noncontiguous (6 to 16%) injuries.1,3,10,11 Subaxial cervical spine injuries involving multiple levels are more common in children 8 years of age or older than in younger children.12 This highlights the need to survey the entire vertebral column in every child who presents with a spinal column injury.
60.1.5 Injury Pattern
Pediatric spinal column trauma is typically classified according to radiographic findings on plain X-ray and computed tomography (CT). Magnetic resonance (MR) imaging is generally less useful in the initial classification of injury types but has utility in the assessment of stability through a more detailed examination of the associated soft tissues. Based only on skeletal information from imaging with X-rays and CT, four injury patterns are seen in children with spinal column trauma: (1) fracture with subluxation, (2) fracture without subluxation, (3) subluxation without fracture (purely ligamentous injury), and (4) spinal cord injury without radiographic abnormality (SCIWORA).13 Although an oversimplification, there is a rough correlation with the degree of instability inherent in this classification: fractures with subluxation are generally unstable; fractures without subluxation may or may not be unstable, depending on the amount of associated ligamentous injury; subluxation without fracture is by definition unstable, although the instability may not manifest immediately but instead later, as a delayed or progressive kyphotic deformity. SCIWORA is a very rare form of radiographically occult instability that is not demonstrable on imaging with conventional X-rays or CT. In the vast majority of cases, however, radiographic abnormalities are seen on MR imaging. Immobilization measures should be undertaken to prevent possible recurrent, progressive, or catastrophic injury.
Age profoundly affects the injury pattern within the pediatric population. In general, younger children (0 to 9 years) are most likely to have a purely ligamentous injury or SCIWORA, whereas older children (10 to 17 years) are more likely to have fractures with or without associated subluxation.1,3,14 In children with cervical spine injuries, the incidence of fracture is approximately 20 to 25% in the 0- to 8-year range versus 70 to 80% in children older than 13 years.15 Despite these numbers, it is important to note that although vertebral column fractures are less frequent in younger children, they are not uncommon; in some series, the incidence of fracture in children 0 to 9 years old with spinal column trauma has been reported to be as high as 50%.1,3
In addition to the influence of age on the pattern of spinal column injury, there is a significantly higher incidence of neurologic injury in younger versus older children. The degree of neurologic compromise has been shown to correlate with the presence of subluxation. As such, children having fractures without subluxation tend to have fewer neurologic injuries (20 to 25%) than those with fracture and subluxation or subluxation without fracture (purely ligamentous injury) (> 50%).3 Furthermore, among children with spinal cord injury (SCI), younger children are more likely to experience severe SCI.1,3 In most case series of pediatric spine trauma, the numbers of patients who are neurologically intact and those with SCI are approximately equal.1,3–6
60.2 Embryology
Embryogenesis of the spinal column occurs in six separate but overlapping phases: (1) gastrulation, (2) condensation, (3) reorganization, (4) membranous proliferation, (5) chondrification, and (6) ossification. Congenital malformations are generally the result of errors during one of the first five phases, whereas the ossification phase is the most important with regard to the response of the vertebral column to trauma and its subsequent management.
Gastrulation occurs during the second week of embryogenesis, when the embryo is converted from a two-layered to a three-layered structure containing ectoderm, mesoderm, and endoderm. This process begins with formation of the primitive streak at the caudal end of the embryo. As the primitive streak subsequently regresses, mesodermal cells from the Hensen node ingress through a primitive pit to form the midline notochord.16,17 The elongating notochord is flanked on each side by newly developed somitic mesoderm; together, these tissues ultimately form the axial skeleton (the intervertebral disks and vertebrae, respectively).
Once in final position, the somitic mesoderm aggregates into discrete, bilaterally paired blocks of tissue, called somites, in a process termed condensation. Both the notochord and somite pairs develop and mature in a rostral-to-caudal direction. The maximum number of somites in the human embryo is generally reported to be 42 to 44, although no more than 38 or 39 are required for formation of the axial skeleton.18
Reorganization involves the development of two segments within each somite: a ventral sclerotome, which contributes to the axial skeleton, and a dorsal dermomyotome, which becomes the dermis and subcutaneous tissues of the back and the dorsal trunk musculature.
The membranous phase of spinal column development begins during the fifth week of embryogenesis. Sclerotomal cells from each somite pair migrate toward the midline and surround the notochord ventrally and the neural tube dorsally. The vertebral centrum is formed by the merging of these cells on either side of the notochord and becomes visible in human embryos shortly thereafter. Craniocaudal polarity begins during somite formation and is restricted to the sclerotomal portion of the somite.19 The dorsal arch of each vertebra appears to be exclusively derived from the caudal, more densely packed, half of the sclerotome. Most evidence tends to support the concept of resegmentation, in which each somite pair directly contributes to the formation of two adjacent vertebral centra.20,21 This accounts for the observation that each spinal nerve passes caudal to its corresponding pedicle.
Chondrification centers appear within the sclerotomes during the sixth embryonic week. The annulus of the intervertebral disk is produced from the condensation of cells from perinotochordal tissues, while physaliphorous cells of the notochord form the central nucleus pulposus. The anterior longitudinal ligament and posterior longitudinal ligament also form during chondrification.
The ossification phase begins in the eighth week of embryogenesis and continues until adolescence.22 It is the most relevant phase of spinal embryogeneis with regard to the ability of the pediatric spine to withstand traumatic forces. The total number of ossification centers that form within each vertebral segment is still the subject of debate; however, most authors argue for three primary ossification centers: one for the vertebral centrum and one on each side for the dorsal vertebral arch. The ossification centers on each side of the dorsal arch subsequently form three progressively independent zones of ossification: one each for the pedicles, lamina, and transverse processes. The junction of the ventral and paired posterior ossification centers occurs at the neurocentral joint. Importantly, the neurocentral joint lies within the vertebral body, not at the junction of the pedicle with the body. Thus, the centrum, combined with the more ventral portions of the dorsal ossification centers, gives rise to the vertebral body. The terms centrum and vertebral body are therefore not strictly synonymous.
Cartilaginous zones subsequently appear cranial and caudal to the ventral ossification center at the cartilaginous end plates. The ring apophysis, a C-shaped structure, forms at the periphery of the cartilaginous end plates, between the developing intervertebral disk and the expanding ossification center of the vertebral centrum. By 11 to 14 years of age, foci of ossification appear within the ring apophysis and become confluent by 15 years of age, forming the radiographic ring. The ring apophysis fuses with the vertebral centrum during middle to late adolescence. This is particularly relevant since during childhood and adolescence the unfused ring apophyses may fracture in response to trauma, which can simulate a herniated disk with nerve root impingement, but is less likely to respond to conservative therapy.23
The CVJ develops from the four occipital sclerotomes (somites 1 through 4) and the first and second cervical sclerotomes (somites 5 and 6). The occipital bone, clivus, and occipital condyles form from the four occipital sclerotomes (somites 1 through 4), with the condyles, paracondylar processes, and ring of the foramen magnum arising from the fourth occipital sclerotome (somite 4). The atlantoaxial complex forms from the fourth occipital sclerotome (somite 4) and the first and second cervical sclerotomes (somites 5 and 6).
Chrondrification of the CVJ begins by embryonic day 45, and the cartilaginous anterior arch of C1 appears by day 50 to 53.23 The anterior arch of the atlas is not ossified in 80% of newborns, and ossification is completed between 6 and 24 months postnatally. A synchondrosis is occasionally present in the anterior arch midline and should not be confused with a fracture.
The odontoid process separates from the atlas between the sixth and seventh weeks of embryogenesis.24 Fusion of the odontoid process to the axis body at the dentocentral synchondrosis begins at about 4 years of age and is completed by 8 years; however, it has been known to remain visible on lateral radiographs in 50% of children up to 11 years old.25 A common radiographic misinterpretation is that of the dentocentral synchondrosis as a type II odontoid fracture; however, the location of the synchondrosis is actually below the anatomical base of the odontoid. Injury to this area in young children is usually not technically a fracture, but rather epiphysiolysis of the dentocentral synchrondrosis. The apical ossification center is generally visible by age 3 and fuses to the rest of the odontoid process by age 12. The apical synchondrosis can often be misinterpreted as a type I odontoid fracture. Failure of ossification of the apical synchondrosis is termed ossiculum terminale persistens (or os avis) and is not considered to be a pathologic condition.
60.3 Biomechanics
60.3.1 General Principles
The developing spine has several unique anatomical and physiologic properties that result in biomechanics that are significantly different from those of the mature adult spine. The main biomechanical difference between the pediatric and adult spine is that the developing spine is inherently more malleable to physiologic and pathologic forces and thus can withstand considerable movement between vertebral segments without damage, but at the cost of providing less protection to the underlying spinal cord.
The developing spine undergoes a biomechanical maturation at approximately 8 to 9 years of age, after which it more closely resembles that of adults.14,25–27 Evidence indicates that this transition takes place more abruptly in the upper cervical spine than in the lower cervical or thoracolumbar spine.3,6,27 The single most important concept in spinal biomechanics is that of stability. Instability has been defined by White and Panjabi as “loss of the ability of the spine under physiologic loads to maintain its pattern of displacement so that there is no initial or additional neurological deficit, no major deformity, and no incapacitating pain.”28 Current models to predict injury have important limitations, particularly with respect to the pediatric spinal column. Few studies have directly investigated the biomechanics of the developing spine; therefore, many assumptions regarding its biomechanics are extrapolations from adult clinical and laboratory data, and these are viewed as particularly inaccurate with regard to the CVJ.
Despite these limitations, several general biomechanical premises and predictions relating to the developing pediatric spine have been suggested.29 First, the malleability of the developing spinal column is increased by its more elastic ligaments, so that it is less likely to sustain fractures and ligamentous rupture when subjected to extrinsic forces but more likely to result in SCIWORA. Second, because it is more malleable, the pediatric spine undergoes more intervertebral displacement in response to extrinsic forces, affording less protection to the spinal cord, making SCI possible in the absence of fracture or subluxation. Third, the most unstable region of the developing spinal column is the upper cervical spine. Trauma to this region should result in fewer fractures but more severe SCI in young children than in older children and adults. Finally, the pattern of SCI in older children (more than 8 years old) should closely resemble the patterns seen in adults, with lower cervical injuries being more common.
60.3.2 Craniovertebral Junction
The occipitoatlantoaxial (O–C2) complex functions as the biomechanical unit of the CVJ. Interacting together as a single unit, the occipitoatlantal (O–C1) and atlantoaxial (C1–C2) joints control movement of the head in relation to the subaxial spine. The atlas (C1) functions as a biconcave washer wedged between the occipital condyles and the superior articulating facets of the axis (C2). The articulation between the occipital condyles and C1 (O–C1) is cup-shaped in the sagittal plane and tilted medially in the coronal plane. This orientation allows up to 20 degrees of flexion–extension and 8 degrees of lateral bending at the joint.28 Further extension at O–C1 is limited by contact of the posterior arch of the atlas with the opisthion at the base of the skull.30 Lateral bending of more than than 8 degrees in either direction is inhibited by the contralateral O–C1 joint capsule. The oblique orientation of the O–C1 articulation precludes all but 4 degrees of rotation to either side at this joint.31,32
Although essentially no lateral bending occurs at C1–2, the articulation between the lateral masses of C1 and C2 is biconcave in the sagittal plane and tilted laterally in the coronal plane, allowing a large degree of axial rotation centered on the odontoid process.28,33 Rotation at C1–2 ranges from 32.2 to 47 degrees to one side and accounts for up to 60% of the rotation of the entire cervical spine.34–36 The first 20 to 30 degrees of head rotation occurs predominantly at the C1–2 joint, during which C1 moves independently of C2. As head rotation passes beyond 30 degrees, the C1–2 joint capsules and alar ligaments begin to reach the limit of their ability to stretch and C2 begins to follow C1 in rotation, but C1 continues to rotate faster than C2.31,34 The maximal degree of rotation between C1 and C2 is reached at approximately 47 degrees. Subsequent head turning is then accomplished by rotation of the subaxial spine. It is important to note that axial rotation is coupled to other motions within the CVJ. Maximal rotation is associated with 1.7 to 4.9 degrees and 3 degrees of lateral bending to the opposite side at O–C1 and C1–2, respectively.36 Rotation is additionally coupled to extension, with up to 13 degrees of extension at O–C1 and 6 degrees at C1–2. Finally, because of the biconvex articulation between C1 and C2, downward translation occurs at this joint with rotation. Before spinal maturation, there is exaggerated motion at the articulation between the anterior arch of the atlas and the odontoid process. Flexion–extension of up to 20 degrees can occur at C1–2 because of physiologic overriding of the anterior arch of C1 along the dorsally curved odontoid process, particularly in very young children (younger than 3 years old), in whom the apex of the odontoid process is not yet ossified. The normal atlantodental interval (ADI) is less than 5 mm in children before spinal maturation (less than 8 years), compared with a normal value of less than 3 mm in adults. Twenty percent of normal children younger than 8 years have an ADI between 3 and 5 mm.25
Stability of the CVJ is provided by several major elements: the cup-shaped O–C1 articulation and its associated capsular ligaments, the tectorial membrane, the alar ligaments, the odontoid process, and the transverse portion of the cruciate ligament.37–39 The transverse ligament is the thick horizontal portion of the cruciate ligament and is the primary constraint to posterior translation of the odontoid into the spinal canal. The tectorial membrane is a continuation of the posterior longitudinal ligament and functions to hold the body of C2 firmly to the clivus and anterior rim of the foramen magnum. Flexion at the CVJ is limited initially by contact between the basion and odontoid process. The tectorial membrane limits additional flexion as the basion slides forward and downward over the odontoid tip. Extension at the CVJ is also limited by the tectorial membrane and through contact between the opisthion and the posterior arch of the atlas. The paired alar ligaments, which run obliquely from the posterolateral surface of the odontoid to the medial occipital condyles, impart stability to lateral bending and rotation.34 Studies have demonstrated that disruption of the tectorial membrane results in excessive flexion–extension and longitudinal displacement (> 1 cm) of the basion from the odontoid process. Compromise of the alar ligaments results in increased lateral bending and rotation. Frank occipitoaxial subluxation occurs when both the tectorial membrane and alar ligaments are disrupted.39 In vivo clinical MR imaging data in children have demonstrated that involvement of the tectorial membrane is a critical threshold for instability in injuries involving the CVJ.40 Larger paraspinal muscles have also been shown to contribute to the stability of the CVJ and are frequently involved in isolated or soft-tissue injuries.40,41
The CVJ is the area of the pediatric spine that is most vulnerable to injury and potential instability. Injury to the O–C2 complex is the most common cervical spine injury in children less than 10 years of age.42 Several factors contribute to this susceptibility. First, articulations in the CVJ of a child are anatomically more predisposed to motion than those in adults. Second, as in the subaxial spine, the major stabilizing ligaments and paraspinal muscles are less developed and more elastic. Third, before approximately 8 years of age, the dentocentral synchondrosis between the odontoid process and the body of C2 is unfused. Generally, odontoid fractures in young children are actually epiphysiolysis of the growth plate at the dentocentral synchrondrosis.43 Congenital or chronic anomalies of the CVJ, such as os odontoideum and occipitalization of the atlas, can result in vulnerability to injury despite relatively minor trauma. The fulcrum of the cervical spine in infants and young children lies within the O–C2 complex as a consequence of the relatively large head size and short neck. Injuries to this region in children are mostly ligamentous or soft-tissue disruptions, rather than fractures, and are highly unstable.
60.3.3 Subaxial Spine
The stability of the subaxial spine is determined by (1) the ability of the vertebral bodies, facet joints, and intervertebral disks to withstand compressive forces and (2) the ability of spinal ligaments to withstand tensile forces.44,45 The pediatric subaxial spine is inherently more mobile than the adult spine, allowing considerably more movement at each vertebral segment in response to extrinsic forces. Several factors account for this hypermobility, including: (1) anterior wedging of the immature vertebral bodies, facilitating forward movement of adjacent motion segments14,25,26,46,47; (2) shallow and more horizontally oriented facet joints, particularly in the upper cervical spine, requiring less force to elicit translation or flexion–extension14,25,26,46,47; (3) absence of the uncinate process in children less than 10 years old, resulting in less restriction to lateral and rotational movements in the subaxial cervical spine47; (4) more elastic spinal ligaments and joint capsules46,47; (5) intervertebral disks that are more expansile in the longitudinal axis as a result of a higher water content48,49; (6) epiphysiologic growth zones at the vertebral end plates that split readily from the vertebral centrum under moderate shear forces50; and (7) underdeveloped paraspinal muscles and a disproportionately large head that predispose to large degrees of motion in response to flexion–extension forces.
Within the subaxial spine, the upper cervical region is particularly hypermobile and is most susceptible to injury from flexion forces. The fulcrum for maximal flexion–extension in the subaxial cervical spine is at C2–3 in infants and young children and moves progressively caudal with increasing age until spinal maturity is reached.47,51,52 By 5 to 6 years of age, the fulcrum is at C3–4, whereas in adolescents and adults, it is located at C5–6. The elasticity of the interspinous ligaments in the developing spine is inversely proportional to age, accounting for the higher incidence of radiographic pseudosubluxation in the upper cervical spine of young children, most commonly at C2–3, but also seen at C3–4.53,54 Normal sagittal intervertebral translations of up to 4 mm can occur at C2–3 in children less than 12 years old. A 24% incidence of moderate to marked C2-on-C3 subluxation has been demonstrated in children between 1 and 7 years old, whereas 46% of children less than 8 years old had 3 mm or more of anterior–posterior motion of C2 on C3 on flexion–extension studies.25 Greater degrees of subluxation in the upper cervical spine should be considered unstable, and the normal upper limit of translation is 3 mm in the remaining subaxial spine. Children 12 years of age and older should not have physiologic motion greater than 3 mm.
60.4 Initial Evaluation and Treatment
Spinal column injury should be suspected in any child after trauma from an appropriate mechanism of injury (i.e., motor vehicle accident, fall, sporting accident) or with posttraumatic neurologic deficit. Initial management in the field and emergency department should always focus on maintenance of the airway, breathing, and circulation. The maintenance of adequate perfusion and blood oxygen levels immediately following trauma is of the utmost importance and may be more beneficial than any subsequent pharmacologic or surgical intervention.
During initial resuscitation efforts, appropriate immobilization of the patient’s spine should be employed to prevent additional injury. In older children and adolescents, standard back board and cervical spine immobilization techniques can be used. In young children, however, the disproportionately large head may result in flexion of the cervical spine when the patient is immobilized in the supine position.55 This should be avoided by bolstering the torso with padding or using a specialized board with a recess for the occiput. Use of a cervical collar is often problematic in infants; they are often best immobilized with the head and torso temporarily taped to the board. An underappreciated cause of avoidable distraction following immobilization is an inappropriately sized cervical collar. Log rolling should be used to move and position children with suspected spine injuries for diagnostic studies.
Several mechanisms of injury are significantly associated with spine injuries and should prompt complete screening radiographs. These include significant fall, pedestrian-versus-motor vehicle accident, abdominal lap seat belt injury, ejection from a motor vehicle, and suspected child abuse. Additionally, any child presenting with neck or axial back pain, neurologic deficit, or reduced mental status and an appropriate traumatic mechanism should be assumed to have a spinal column injury until it is proved otherwise. Several cutaneous findings that are associated with spinal column injuries include seat belt bruises of the abdomen and neck, subcutaneous emphysema, displacement of the spinous processes, and tire tracks across the back. Given the high incidence of both multiple contiguous and noncontiguous spinal column injuries in several series, the discovery of a spine injury at any level should trigger radiographic screening of the entire spinal column.3,10,11 Once a determination has been made that a spinal column injury is present, the same principles that apply to adult spine trauma are employed: (1) a determination of biomechanical instability, (2) closed or open reduction (and decompression) if necessary, and (3) appropriate spinal immobilization (external or internal).
60.5 Diagnostic Imaging
The combined use of common imaging modalities, including plain radiographs, targeted fine-cut CT, and MR imaging allows the clinician to identify both osseous and soft-tissue injuries and to make a determination regarding resulting instability. Of particular importance in the pediatric population is obtaining an accurate diagnosis while minimizing the exposure of the developing child to ionizing radiation. Ultimately, the treatment of pediatric spinal column trauma is based upon the neurologic status of the patient, diagnostic imaging results, and degree of maturation of the spinal column.
Plain radiographs are the mainstay of initial diagnostic imaging in any child with suspected spinal column trauma. Although specific guidelines exist for the radiographic assessment of adults with suspected spinal column trauma, there is still controversy regarding the appropriate indications for screening radiographs in children. Proposed pediatric protocols are usually aimed at ensuring that clinically significant injuries are not missed, while simultaneously limiting exposure to ionizing radiation. A prospective multicenter study by Viccellio et al in 2001 used the National Emergency X-Radiography Utilization Study (NEXUS) criteria to evaluate for cervical spine injury in children younger than 18 years.56 The authors defined five high-risk criteria, including: (1) midline cervical tenderness, (2) evidence of intoxication, (3) altered level of alertness, (4) focal neurologic deficit, and (5) painful distracting injury. If any one of these criteria was met, the patient was considered high-risk; however, if none was met, they were low-risk. Of 3,065 children in the study, 603 (19.7%) were defined as low-risk, none of whom ultimately had a documented cervical spine injury. Conversely, there were 30 (0.98%) cervical injuries in the group of children considered to be high-risk. The results of this study clearly demonstrate that application of the NEXUS criteria to children reduces the need for screening cervical spine radiographs by almost 20% without resulting in any missed injuries.
The necessity and utility of open-mouth plain radiographs in pediatric trauma patients have been questioned.57,58 Current evidence suggests that the open-mouth odontoid view may not be routinely needed in children less than 5 to 9 years old.57,58 There is agreement that open-mouth views should be attempted in children 9 years of age and older. Conversely, dynamic imaging is of particular importance in the assessment of pediatric spinal column trauma. Ligamentous injury is more common than osseous injury in children and cannot be excluded based on the demonstration of normal bony anatomy on static radiographs; therefore, flexion–extension imaging should be obtained for responsive and cooperative patients during initial screening if spinal column trauma is suspected. In children unable to tolerate this, ligamentous injury may be assessed by using MR imaging with fat-suppressed sequences, or dynamic imaging may be attempted in a delayed fashion. Because the sensitivity of MR imaging to detect edema associated with ligamentous injury is reduced after 48 hours from spinal column trauma, the study should be obtained within this time interval in order to be diagnostic.59 Ligamentous injury may also be assessed in a delayed fashion in unresponsive patients by using dynamic imaging under direct fluoroscopic guidance. In such patients, MR imaging should still be considered to rule out an occult compressive lesion before dynamic imaging is obtained because the patient’s neurologic examination cannot be followed reliably during flexion and extension.
MR imaging should be obtained in all children with a neurologic defict and should also be considered before closed reduction and external immobilization or operative reduction and fixation to assess the degree of canal compromise. If the patient has previously implanted ferromagnetic instrumentation or intra- or paraspinal bullet fragments, CT myelography can be substituted for MR imaging to exclude compressive lesions.
CT should be used judiciously in the pediatric population in order to minimize exposure to ionizing radiation. It is not recommended as a means to clear the entire cervical spine, but its targeted use is helpful in evaluating osseous structures that are poorly visualized on plain radiographs. Many radiographic determinants of spinal column instability are difficult to assess accurately on plain radiographs, and in these cases further imaging with CT may be warranted. If an injury is suspected on plain radiographs, fine-cut CT should be obtained through the suspicious and adjacent levels to better define the anatomy, to survey for adjacent fractures, and to detect areas of incomplete ossification that may influence operative management.
60.5.1 Radiographic Determinants of Craniovertebral Junction Instability
Plain radiographs provide the most practical method of determining instability at the CVJ. Several radiographic criteria have been developed based on distances between osseous structures as seen on X-ray imaging (▶ Fig. 60.1). When visualization of these structures is poor, accurate assessment of CVJ instability may require CT of the O–C2 complex. It is important to note that extrinsic forces, such as positioning and immobilization, in highly unstable CVJ injuries may influence the anatomical relationships of the bony elements following injury. In children, these forces tend to be flexion due to the large head size in relation to the torso and distraction from a cervical collar. Additionally, plain film measurements have traditionally exhibited variation in response to differing magnification from discrepancies in film-to-target distances. The Powers ratio, Lee X line, and C1–2:C2–3 ratio were originally designed as dimensionless measurements to address this issue. However, with the widespread use of digital radiographic collection and processing systems, this problem has been mitigated. When instability of the O–C2 complex is suspected, it is imperative to use the following criteria in combination to attain a complete and thorough assessment. Only one positive finding should be considered necessary and sufficient for a diagnosis of CVJ instability.
Occipital Condyle–C1 Joint Interval
The O–C1 joint interval (CCI) is the distance between the occipital condyle and the superior facet of C1 (▶ Fig. 60.1a). Based on plain films, Kaufman et al found that this interval in children should not exceed 5 mm between any opposing points of the joint articulation on lateral or anteroposterior views.60 Interpretation of the CCI on X-ray is nearly impossible, however, because of superimposition of the mastoids and the imperfect overlap of the two O–C1 joints.
This difficulty is overcome with thin-cut CT. Using this modality, Pang et al established 4 mm as the upper limit of normal for the CCI in children.61,62 In a series of 89 normal children, the mean combined CCI for all 178 joints was 1.28 ± 0.26 mm, with no single CCI larger than 1.95 mm.61,62 Sagittal–coronal variation of the CCI is approximately 12%, and left–right variation is less than 3%. No individual measurement in any projection exceeded 2.5 mm. By using 4 mm as the upper limit of normal for the CCI, Pang et al subsequently demonstrated that at least one and usually both O–C1 joints were separated in all children with proven atlanto-occipital dislocation (AOD) regardless of Traynelis classification.63 These children often had horizontal displacement or fractures of the O–C1 joint in addition to longitudinal separation. Coronal reconstruction through the occipital condyles demonstrates the true extent of AOD, with asymmetry suggesting a rotary component to the displacement. The CCI is the only measurement that directly measures the O–C1 articulation and is considered by most authors to be the single most reliable diagnostic criterion for O–C1 instability.
Fig. 60.1 Radiographic determinants of craniovertebral junction instability. (a) Occipital condyle–C1 (O–C1) joint interval (CCI). The mean CCI is determined by measuring and averaging the distance across the joint space at four equidistant points (white arrowheads) along the occipital condyle and C1 superior articulating facet. The CCI is considered by most authors to be the single most reliable criterion of O–C1 instability, and a value ≥ 4 mm is considered abnormal.61,62 (b) Dens–basion distance. Measured from the dens (D) to the basion (B), distances of 5 and 12.5 mm are the upper limits of normal in adults and children, respectively.64 A value > 14 mm in children is diagnostic for O–C1 instability.65 (c) Basion–axial interval (BAI). This is the distance between a vertical line drawn through the basion and the posterior axial line (PAL; white arrowheads); it is usually < +12 mm (anterior to the PAL) in normal children.66 (d) Powers ratio. The ratio is determined by dividing the distance between the basion (B) and the midpoint of the posterior arch of C1 (C) by the distance between the opisthion (O) and the midpoint of the anterior arch of C1 (A).68 Normal values typically are 0.77 ± 0.09, with a value > 1 indicative of anterior atlanto-occipital dislocation (AOD).29,69
(e) Lee X line. Lines from the basion (B) to the midpoint of the C2 spinolaminar line (C2SL) and from the opisthion (O) to the posteroinferior body of C2 are drawn.70 The first line should tangentially intersect the posterosuperior dens, and the second should intersect the highest point on the C1 spinolaminar line. At least one intersection should be present; if neither is present, AOD should be suspected. This is of limited utility in children because it requires a fully formed odontoid process to be accurate. (f) Atlantodental interval (ADI). The distance between the anterior arch of C1 and the ventral surface of the dens (white arrowheads) should be < 3 mm in adults and 5 mm in children older than 8 years.28,54,71,72 Values greater than these suggest transverse ligament compromise. (g) C1–2:C2–3 ratio. This is obtained by dividing the shortest distance between the posterior arch of C1 and the spinous process of C2 by the shortest distance between the spinous processes of C2 and C3 (white arrowheads).40 Damage to the tectorial membrane may result in ratios > 2.5.
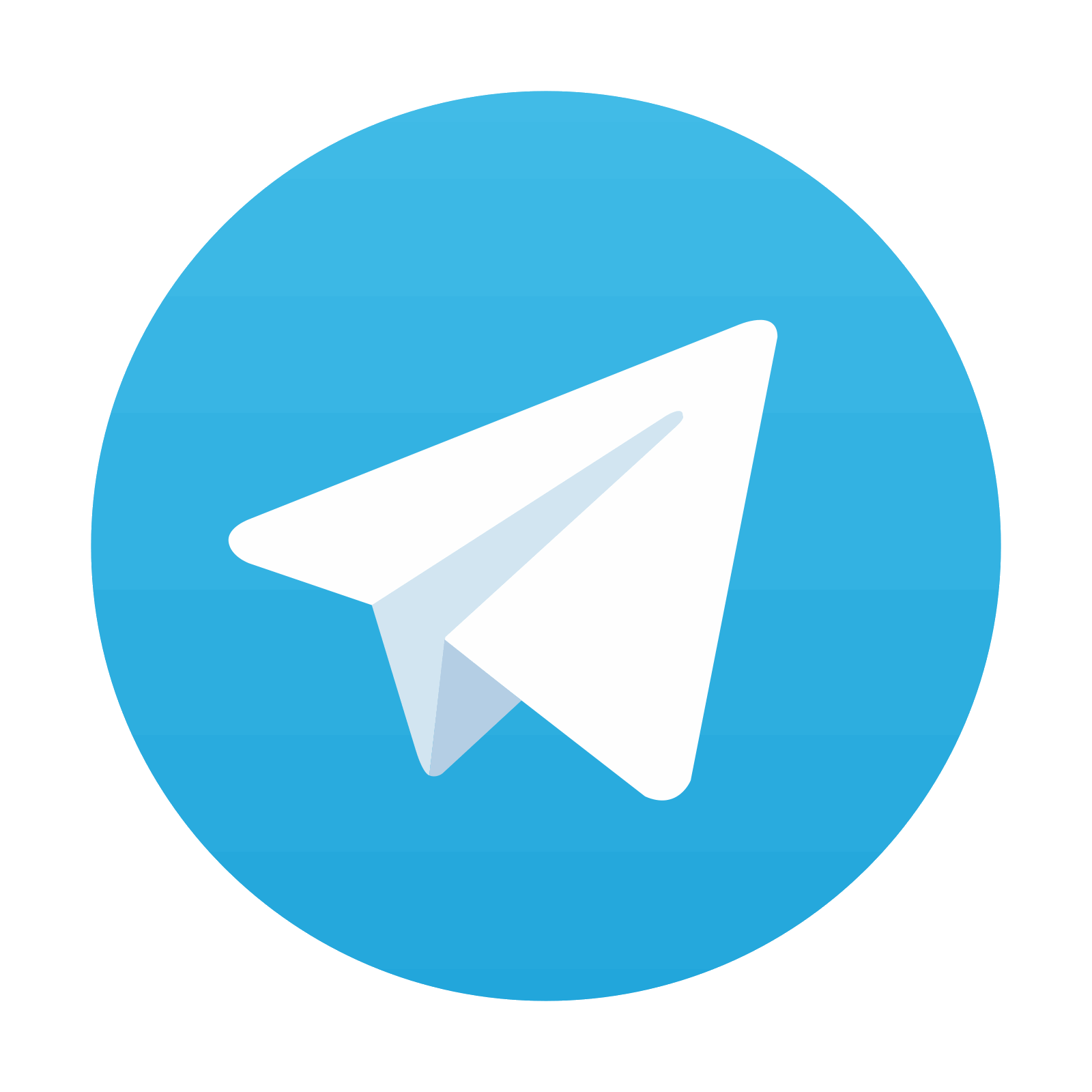