CHAPTER 248 Principles of Radiation Therapy
Basic Principles of Radiation Therapy
Cellular Effects of Radiation
Radiation therapy uses ionizing radiation, which means that the radiation’s energy is sufficient to remove an electron from the outer shell of an atom (more than 124 electron volts).1 In clinical therapy, photon radiation is most commonly generated by linear accelerators or gamma-ray sources that produce a range of ionizing photon energies. When a high-energy photon interacts with an atom, the atom becomes unstable and is more apt to react with surrounding atoms. With this simple model of ionizing radiation as background, and remembering that in many ways standard radiation sources or beams act like visible light, we can explain the effects of radiation on cells.
Evidence supporting that DNA is the most critical cellular target of radiation includes (1) genetic damage and specific chromosomal abnormalities after irradiation are correlated with cell death, (2) defects in cell DNA repair mechanisms increase radiosensitivity, and (3) selective irradiation of the cell nucleus as opposed to cytoplasm has mimicked the overall effect of radiation on cells.1 This basic effect of irradiation on DNA and the cell’s subsequent repair underlie the observed biologic effects of conventional fractionated radiotherapy treatment.
There is an active interest in increasing application of particle therapy, specifically protons. As opposed to behaving like a light wave, protons deposit their energy at a specified depth over a more tightly defined area, or Bragg’s peak. The pattern of energy distribution of particle therapy (electrons and protons) compared with photon therapy (x-ray) is shown in Figure 248-1. Particles are charged and have physical properties that cause direct damage to the DNA (rather than through reactive water molecules). Their clinical advantage over photon therapy is in less dose to normal tissues, which may be especially important in children who are at risk for radiation-induced malignancy. Electron therapy is readily available on all linear accelerators and has been used clinically for decades; however, this modality is rarely used in central nervous system (CNS) malignancies. Proton therapy requires a multimillion dollar machine called a cyclotron and thus remains less commonly available and of uncertain, albeit promising, importance in years to come.2,3
Therapeutic Ratio for Radiation
Biologic Selectivity
Normal tissue generally repairs DNA damage more efficiently than neoplastic tissue. Reasons may include metabolic differences, aberrant cell cycle control mechanisms, and inherent cellular differences. Cells require time to repair DNA damage, and the normal cell response to irradiation is to delay cell cycle progression in the G2 phase.4,5 The length of G2 delay correlates with radiation resistance because the cell has more time to repair radiation damage.6
This enhanced repair capacity of normal tissue has been critically exploited by fractionated regimens with low doses of radiation (the total therapeutic dose is broken up into a series of small daily treatments). Because a high percentage of normal cells will repair damage at low doses of radiation and because neoplastic cells are less capable of efficient repair, the concept of fractionation of radiation is supported. Figure 248-2A demonstrates a schematic rendering of the effect of multiple fractions of 2 Gy on both normal tissues and tumor; the more fractions, the greater the therapeutic advantage. These principles were extended to hyperfractionated approaches (two or three small doses per day), although with limited success.7–10
Another clinical observation is that more rapidly dividing normal tissues such as skin and oral mucosa are more acutely sensitive to the effects of irradiation than are late-responding or slowly dividing and nondividing tissues such as nerve tissue.1,11,12 In contrast, late-responding tissues are more sensitive to large doses of radiation. Note that in Figure 248-2B, a large single dose of radiation is capable of greater injury to late-responding normal tissue than to the tumor.11,13 Therefore, to take advantage of the inherent differential cellular capacity to repair sublethal doses of irradiation, multiple small doses of radiation have been recommended. This basic principle supports the biologic advantage of fractionation to selectively protect late-responding nerve tissues.
Technical Selectivity
Therapeutic advantage may also be achieved by depositing more radiation dose in the tumor target than in the normal tissue. A single-photon radiation beam entering a patient begins with a region of lower dose, termed the build-up region,14 and then progressively increases until it reaches the depth of maximal dose (Dmax) that is characteristic of the radiation beam energy (Fig. 248-3). This build-up region spares the skin from the highest doses of radiation. After the radiation beam reaches Dmax, the dose decreases with depth as it is attenuated (see Fig. 248-3). Much like shining a light into a dark field, the light becomes less bright the farther you move away from its origin as it becomes attenuated by air.
To achieve technical selectivity, several beams of radiation can be added together from several directions (Fig. 248-4). A single beam of radiation entering a patient is shown in Figure 248-4A. In this figure, a group of same dose, or isodose lines, is labeled as a percentage of maximal dose. The percentage of maximum decreases with depth owing to attenuation. When we put a tumor at the intersection of several beams of radiation oriented at divergent angles, the isodose lines are as shown in Figure 248-4B to D. Note that the tumor receives the highest dose, and areas outside the tumor now receive progressively less dose. As multiple beams are added together, the isodose lines become densely concentrated at higher percentages of maximum, as shown in Figure 248-4D. In considering these physical characteristics of radiation beams, these examples illustrate the capacity of multiple radiation beams to deliver a higher percentage of the radiation dose to the tumor, with progressively smaller areas of normal tissue receiving significant doses.
In addition to the additive effects of multiple converging beams of radiation on the target, each beam may be selectively filtered in the plane of its delivery. The intensity of light could then be altered at different areas in a given field of radiation. If we could control this factor as a radiation beam was delivered, we could then further shape the radiation beam. This is accomplished practically by a series of moving leaves that are computer controlled and move across a radiation field during treatment. This leaf-moving device is called a multileaf collimator (Fig. 248-5) and is used both to shape static beams and to modulate the intensity of each beam, hence the term IMRT.
Clinical Application of Radiation Therapy
Application of the biologic characteristics and physical characteristics to the clinical discipline of radiotherapy leads us to recognize several parameters of treatment that define both the therapeutic effect on the tumor and the likelihood of normal tissue complications. One important parameter is dose. Dose must be described as total dose as well as dose delivered at each treatment (per fraction). Multiple small doses of radiation generally yield greater biologic advantage,11,13 as illustrated in Figure 248-2A. The small doses are most commonly given once a day and are termed standard fractionation. A second method of delivery is with two or more smaller radiation doses each day, separated by time (4 to 6 hours) to allow for normal tissue repair; this is called hyperfractionation. The total dose and the pattern of treatment delivery (daily or twice daily fractionation) are both critical in determining the effect of radiation treatment on both tumor and normal tissue.11,13,15 More recently, the ability to give a single or a limited number of fractions (hypofractionation) has been studied intensively. In these regimens, the total dose and time over which they are given remains critical in defining the effects.
Another parameter that is important in describing radiation treatment is time.11,13 This has been confirmed clinically in that radiation treatment schemes with gaps or splits in treatment have been less successful than those in which a continuous course has been given.15,16 Furthermore, treatment regimens delivering the same dose over a shorter period have been more effective in treating tumors than those delivered over a longer period.15 Delivering the same dose over a shorter time is termed accelerated fractionation. Hence, treatment descriptions should note the elapsed number of days (time—both treatment and nontreatment days and including gaps in treatment) over which the treatment course was completed.
Another critical parameter for radiation treatment effect is the volume of tissue treated.17 A small area of the brain will tolerate a much larger dose without serious toxicity than will the entire brain. Furthermore, the particular volume that is treated may determine the toxicity; different areas of the nervous system have diverse tolerances, and functional neuroanatomy dictates the observed effect of a toxicity.18
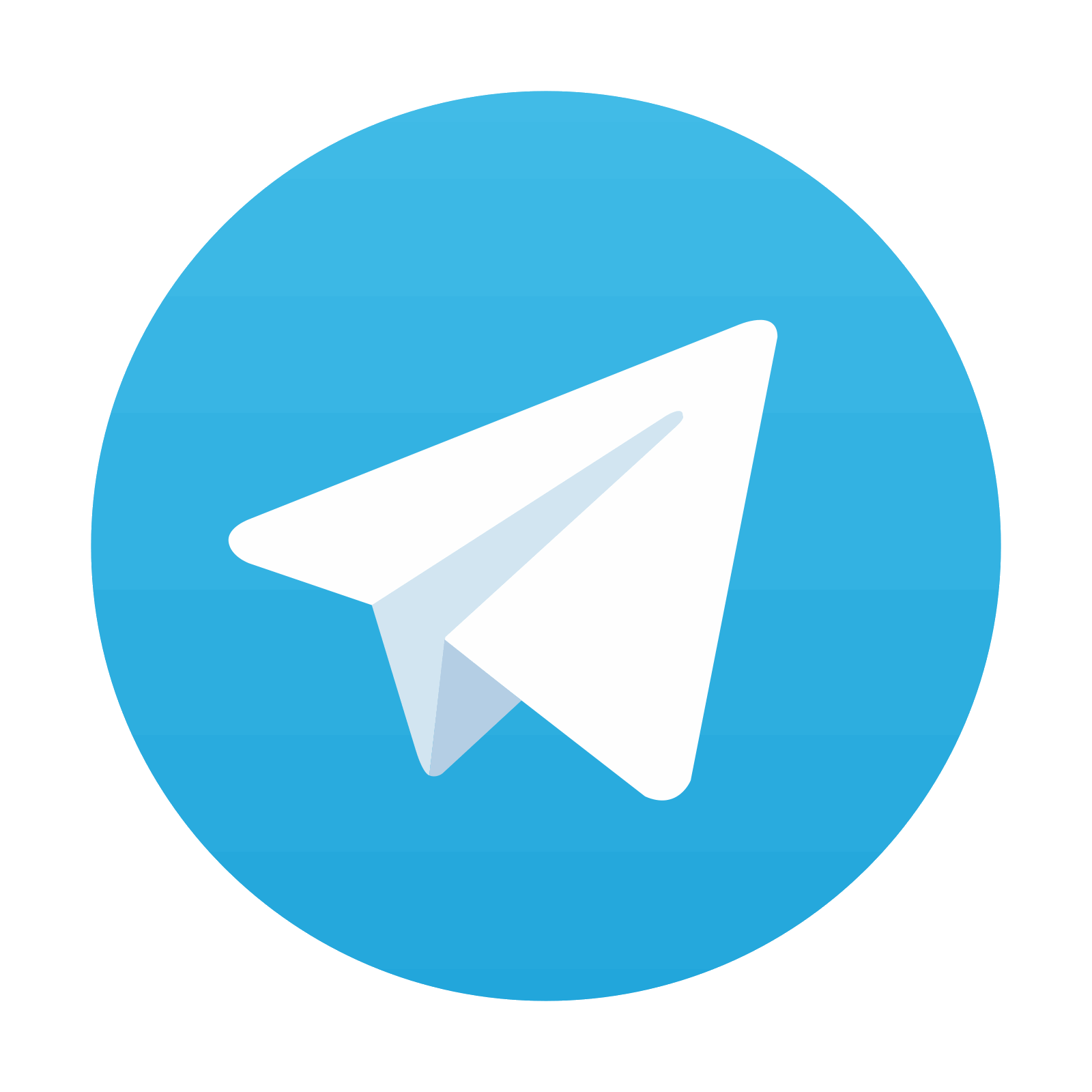
Stay updated, free articles. Join our Telegram channel
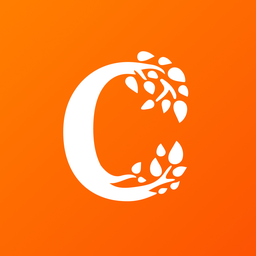
Full access? Get Clinical Tree
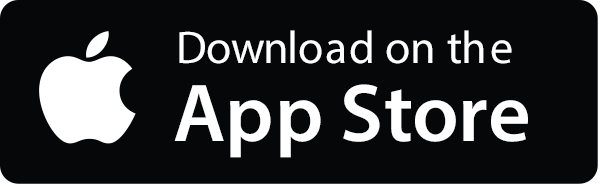
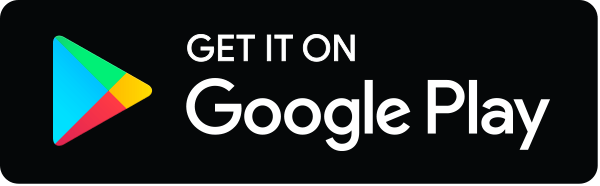