(1)
Asheville, NC, USA
Protein quality control is carried out continuously throughout the cell—in the cytosol, in the endoplasmic reticulum, in the mitochondria and everywhere else. It is an intensive endeavor requiring the participation of multiple sensing, signaling, and degradation pathways. The focus in the last chapter was on the extensive network of molecular chaperones and on the ubiquitin-proteasome system that forms a first line of defense against misfolded and aggregated proteins. In that chapter, several different kinds of protein machines were introduced. Among these were ATP-dependent molecular chaperones and degradases that carry out folding and unfolding and separation and degradation operations on proteins. In this chapter, the autophagic-lysosomal pathway will be described along with another class of protein machines, the molecular motors that transport vacuoles and organelles and their contents to distant locales where they are needed. The autophagic-lysosomal pathway has been the subject of great interest in the field due to its ability to remove damaged organelles and proteins that have become misfolded and aggregated because of mutations or other causes and cannot be handled by the UPS.
Cells belonging to the immune, endocrine, and nervous systems signal extensively. They secrete large quantities of signaling molecules and position numerous receptors on their cell surface. Proteins destined for secretion and membrane localization are translocated into the endoplasmic reticulum where they are modified and subjected to protein quality control . Proteins found to be misfolded by resident chaperones are retranslocated from the ER lumen to the cytosol and degraded by the 26S proteasome by a process termed endoplasmic reticulum-associated degradation (ERAD). One of the widespread characteristics of neurodegeneration is the accumulation of misfolded and aggregated proteins in the ER. That buildup impairs the normal function of the ER, a phenomenon termed ER stress . In response, cells initiate a set of programmatic changes referred to as the unfolded protein response (UPR). Protein quality control in the ER along with the maintenance of mitochondrial health critical for maintaining an adequate energy supply will accompany the exploration of autophagy. The chapter will then conclude with a look at current theories of aging , which have as their main thesis the progressive decline in protein quality control. In that discussion, special attention will be paid to caloric restriction and its ability to promote longevity through a variety of ways.
6.1 Autophagy and the Active Transport of Cargo Are Discovered
By 1963 electron micrographs had revealed the presence in cells of vesicles encapsulated by single or double membranes containing portions of the cytoplasm and organelles in the process of being digested. Earlier, in 1955, Christian de Duve (1917-2013) had discovered the lysosome using a high-speed centrifuge. At a conference on lysosomes, de Duve coined the term “autophagy”, or self-eating, to denote the notion of self-directed degradation of unwanted and damaged cellular components. Today, this disposal route is recognized as playing a major role whenever the ubiquitin-proteasome system is overloaded or cannot handle the materials due to size or composition limitations. Cytosolic materials, misfolded, aggregated, and excess proteins, parts of the ER, the Golgi, and the nucleus, and entire organelles, especially mitochondria, are disposed through autophagic transport and digestion by hydrolytic enzymes in lysosomes.
Autophagy takes one of three forms. In macroautophagy , phagophores are formed that encapsulate the cytoplasmic materials to be degraded. As illustrated in Fig. 6.1 these become autophagosomes when encirclement is completed. These compartments subsequently fuse with lysosomes to form autolysosomes. Autophagic and endocytic pathways converge prior to reaching the lysosomal compartments, and several examples of fusion events of this type captured using electron microscopy are presented in Fig. 6.2. In the second form of autophagy, microautophagy, lysosomes directly engulf small components of the cytoplasm. Lastly, in chaperone-mediated autophagy , substrate proteins are chaperoned one-by-one across the lysosomal membrane.
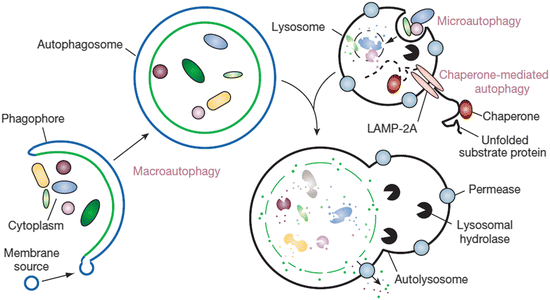
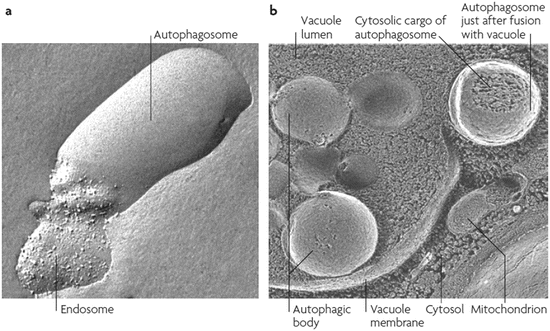
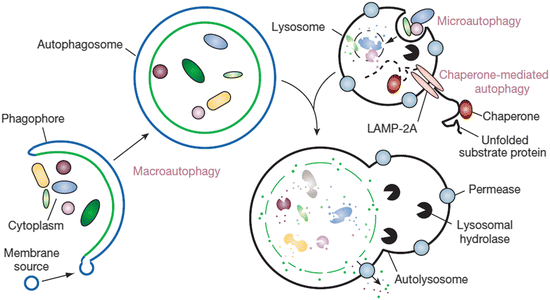
Fig. 6.1
The three different kinds of autophagy. Shown are macroautophagy , microautophagy and chaperone-mediated autophagy (from Mizushima Nature 451: 1069 © 2008 Reprinted by permission from Macmillan Publishers Ltd)
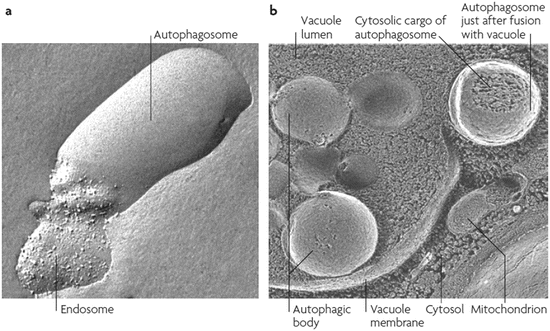
Fig. 6.2
Autophagic events visualized by means of freeze-fractured electron microscopy. (a) Fusion of an autophagosome with an endosome, and (b) fusion of an autophagosome with a vacuole (from Klionsky Nat. Rev. Mol. Cell Biol. 8: 931 © 2007 Reprinted by permission from Macmillan Publishers Ltd)
Macroautophagy is the predominant mechanism, and will hereafter be referred to as autophagy. It can be further broken down into two subtypes. Nonselective autophagy supplies materials for macromolecular synthesis and energy production by recycling the amino acid, free fatty acid, and glucose building blocks. This process is especially valuable during starvation conditions and even takes place under normal conditions, as well. It is estimated that 1 to 1.5 % of cellular proteins are catabolized every hour through nonselective (basal) autophagy. In selective autophagy , specific tagged proteins and organelles are degraded.
Neurons present special challenges due to their morphology. Materials need to be transported over large distances out to axonal and dendritic terminals and back again to the soma, the primary sites of protein synthesis and lysosomal degradation. This requirement necessitates the use of an active transport system. That such a system might exist was first posited by Ramon y Cajal as early as 1928. Evidence in favor of that hypothesis was initially obtained in studies of peripheral nerve regeneration by Weiss and Hiscoe in the years just after the end of World War II, motivated by limb injuries to war veterans. Some years later, with the advent of radiolabelling techniques, the flow of materials out to the periphery from the cell body was directly observed.
By 1980 it was established that two-way flows regularly occurred and with varying rates depending on the the cargoes being shuttled. The cargoes undergoing active transport included organelles, vesicles, cytoskeleton components, receptors, and other signaling proteins. Rates for anterograde transport of neurotransmitters, membrane proteins, and lipids range from 200 to 400 mm/day, while rates for retrograde transport of lysosomal vesicles vary from 200 to 300 mm/day. Mitochondria are transported at reduced rates, 50–100 mm/day, punctuated by stops and reverses designed to uniformly distribute mitochondria throughout the axon. The aforementioned movement processes are referred to as fast axonal transport . That is in contrast to the transport of cytoskeleton components such as microfilaments and microtubules that move at rates up to 100 times slower. The shipping of those cargoes is called slow axonal transport.
6.2 Cargo Is Transported by Motor Proteins along Actin and Microtubule Rails
Active transport is made possible by molecular motors that travel along tracks constructed out of actin filaments and microtubules. Recall that microtubules are polarized structures composed of polymers of α- and β-tubulin. Their fast-growing plus end is directed outward, away from the microtubule organizing center (MTOC), towards the axonal terminal and its slower-growing minus end is directed inward towards the soma and MTOC. Three kinds of motors are utilized in active transport (Table 6.1). Myosins transport cargoes along actin filaments while kinesins and dyneins shuttle cargoes along microtubules. Kinesins mediate the anterograde transport from the cell body out along axons and dyneins move cargo in the opposite, or retrograde, direction back to the cell body. The motor protein s appearing in Table 6.1 belong to large families that carry out ATP-dependent, force-producing mechanical tasks ranging from muscle contraction to ciliary and flagellar movement to walking along rails while conveying cargo.
Table 6.1
Axonal transport and its motor protein s
Motor protein | Type of transport | Rail system |
---|---|---|
Kinesins | Long-distance anterograde | Microtubules |
Dyneins | Long-distance retrograde | Microtubules |
Myosins | Short-distance | Actin filaments |
Myosins, the first of the motor protein s to be studied, have been known for some time because of their role in muscle contraction. Early studies on muscle contraction culminated with the discovery in 1942 that contraction was brought about through the interaction of ATP with two proteins—actin and myosin. A key development in this arena was the development over more than a decade of the sliding filament model and an accompanying picture of force generation by Andrew Fielding Huxley (1917–2012) and others. In their model, actin-myosin linkages form, move, and generate a sliding force when ATP is hydrolyzed by the enzymatic actions of myosin. The discovery of other myosin motors was advanced by the exploration by Pollard and Korn of how amoeboid movement comes about. Those investigations led to the discovery in 1986 of myosin I, initially referred to as an “unconventional” myosin, and in 1993 Rayment solved the first three-dimensional structure of a myosin motor protein.
The discovery of dynein, the first microtubule-associated protein to be found, is intimately connected to explorations of how cilia and flagella move. Those efforts began in the 1950s with the use of electron microscopy to examine the structural core and culminating in 1965 with the isolation of the dynein ATPase by Gibbons and Rowe. The name, dynein, given to the protein by them was taken from “dyne” the Greek word for force. Dyneins are structurally far more complex than either myosins or kinesins, and it was not until 2011 that the three-dimensional crystal structure of its motor domain was solved. Kinesin, the second microtubule-based motor protein , was discovered by several groups in the period from 1982 to 1985. Those efforts were inspired by the desire to find the elusive motor protein responsible for the fast axonal transport of organelles and other cargo. The name given to the new protein, kinesin, was taken from the Greek “kinein”, meaning to move. It is the smallest of the three cargo-transporting motor proteins , and its three-dimensional crystal structure was solved in 1996.
6.3 Structure and Function of the Motor Proteins
Members of the three families of motor protein s are found in multiple kingdoms and carry out a broad spectrum of functions. Of these, five members can be singled out for their prominent roles as cytoplasmic cargo transporters. As illustrated in Fig. 6.3 they each have a pair of heads that make contact with the microtubule and actin rails and contain the ATPase activity. Kinesins and myosins have one ATP binding site per head while dynein possesses a hexameric AAA-ATPase ring with four potential ATP binding sites and two putative regulatory sites. Cargo is carried by the feet of the kinesins and dyneins and in the equivalent location on the dyneins through the dynactin partner complex.
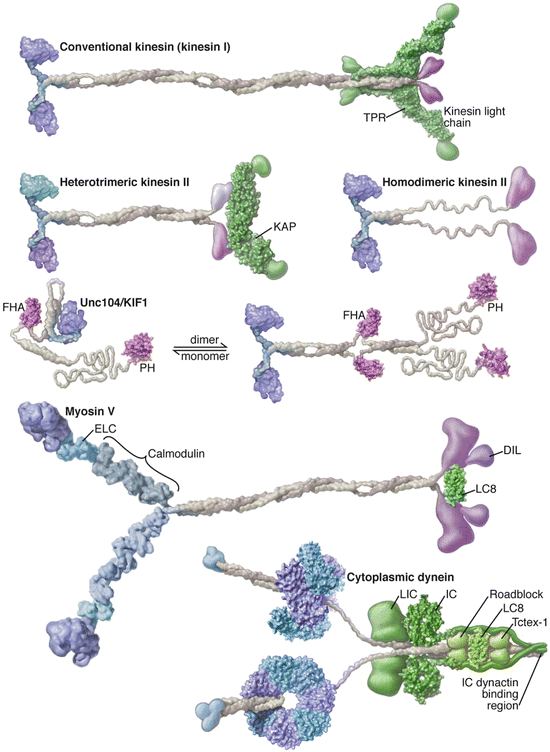
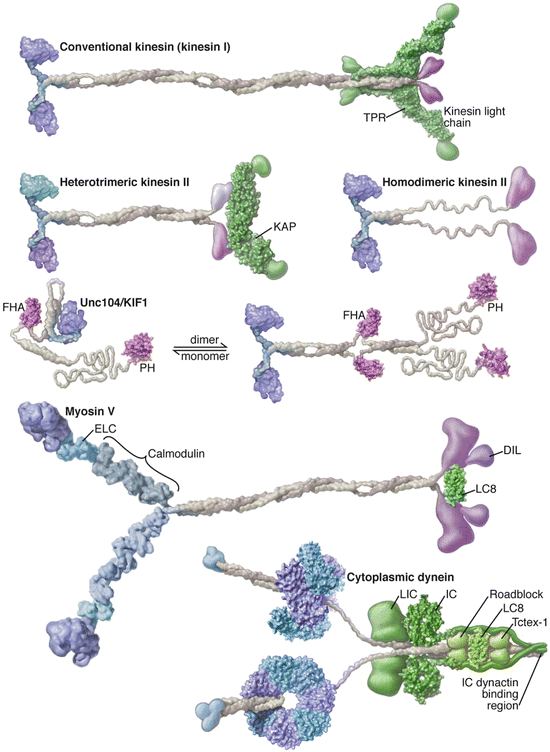
Fig. 6.3
Motor protein s with prominent roles in cargo transport. The kinesins and myosin each contain a head region shown in blue on the left, a long stalk colored grey, and a tail region highlighted in purple on the right. The long structures connecting the myosin heads to the stalk are referred to as the lever arms. The structure of dynein differs in two main ways—it contains a hexameric ring structure connected to a microtubule-binding stalk and in place of the feet it possesses a dynactin-binding region that serves as the interface to the cargo (from Vale Cell 112: 467 © 2003 Reprinted by permission from Elsevier)
Single molecule biophysics methods have been used extensively to probe the structure and dynamics of the walking motors. That includes atomic force microscopy and optical tweezer s . It also includes FRET and the application of quantum dots, fluorescent semiconductor nanoparticles that overcome many of the limitations of conventional fluorophores. Using these single molecule biophysical methods the manner in which these three classes of motor protein move their cargo over actin and microtubule rail system s has been explored.
Kinesins and myosins are stepping motors. As can be seen in Fig. 6.3, kinesin is much smaller than myosin and has been called the world’s smallest biped. In spite of their lack of similarities in physical size, amino acid sequences, and enzymatic activities, the walking motors utilize similar power-generation and walking strategies. Using single molecule biophysics and associated techniques the motor protein s have been found to function in the following manner:
They hydrolyse one ATP molecule for each 8 nm step in the case of kinesins and myosins. Dynein steps are more variable and range up to 20 nm or so. In some instances, a pair of motor protein s may attach to a single cargo, and move that cargo either forward or backward as the need dictates.
The term processive denotes the ability of some motor protein s to take many steps before releasing from the actin filaments and microtubules. These motor proteins may take more than 100 steps before detaching. Homodimeric kinesin 1, myosin V, and cytoplasmic dynein undergo processive movement. However, others such as muscle myosin II move nonprocessively. That is, they undergo isolated movements in which forces are exerted transiently followed by detachment. The movement of both kinds of motor proteins along microtubule and actin tracks is depicted in Fig. 6.4.
Fig. 6.4
Cytoskeletal motor protein form and function. (Aa) Dimeric myosin V processive movement along actin filaments. (Ab) Dimeric myosin II undergoing non-processive movement along actin filaments. (Ac) Kinesin-1 and Dynein movement along microtubules. (B, C) Illustration of the Myosin II and Kinesin-1 power stroke s (from Veigel Nat. Rev. Mol. Cell Biol. 12: 163 © 2011 Reprinted by permission from Macmillan Publishers Ltd)
The movements of the two heads are coordinated, and enable them to move in a hand-over-hand manner. There are three models for the gait—symmetric hand-over-hand, inchworm, and asymmetric hand-over-hand. The asymmetric gait is supported by the majority of the evidence at present. The three ways of walking are illustrated in Fig. 6.5.
Fig. 6.5
Gaits that can be used by walking motor protein s . (a) Symmetric hand-in-hand in which the two heads are completely synchronized. (b) Inchworm in which one head is always in the lead. (c) Asymmetric hand-over-hand, or limping, in which the proteins alternate between two different conformations as they step (from Asbury Curr. Opin. Cell Biol. 17: 89 © 2005 Reprinted by permission from Elsevier)
These three families of motor protein s have important roles in maintaining cellular homeostasis and protein quality control . The short-range, actin-based myosins (e.g., Myosins Va and Vb) move glutamergic and GABAergic receptors as well as recycling endosomes into dendritic spines, and haul transport vesicles into presynaptic terminals. At the same time, the long-range microtubule-based haulers move organelles and granules, and receptors and neurotrophic factors, into and out of axons and dendrites. The kinesins and cytoplasmic dyneins transport mitochondria, RNA granules, endosomes, synaptic vesicle precursors, and NMDA and AMPA receptors. Most importantly, from the viewpoint of protein quality control cytoplasmic dynein hauls misfolded, damaged, and aggregation-prone proteins in autophagic vesicles to lysosomes for fusion and degradation.
The key element in making walking possible is the generation of force, of power stroke s in this case, by the motor protein s . The mechanism underlying this process is remarkably similar to the one used in muscle contraction as identified by Huxley in 1969. Known as the swinging cross-bridge mechanism it has as its main tenet the transduction of chemical energy from ATP hydrolysis into mechanical power strokes. When applied to motor proteins it enables them to move along actin filaments or microtubules. A key observation underlying the mechanism is that small movements generated in the vicinity of the catalytic site are amplified by the motor protein’s light-chain binding region, which acts as a lever arm.
The swinging lever arm mechanism is a more modern name for Huxley’s swinging cross-bridge. The various motor protein s are built on the same broad principles, but differ in a number of ways from one another in their details. These differences are reflected by variations in, for example, precise angles through which the lever arms swing and the step size generated as a result. As shown in Fig. 6.6, the myosin II lever arm swings through 70° thereby generating a 10 nm stroke. Myosin V also swings through 70°, but has a longer lever arm that produces a 20 nm stroke while Myosin VI’s lever arm swings through a full 180° and generates a 30 nm stroke (the latter two not shown). Illustrations of how “walking” is generated through cycles of ATP/ADP binding and release by the catalytic heads of myosins, kinesins, and dyneins are presented in Fig. 6.4.
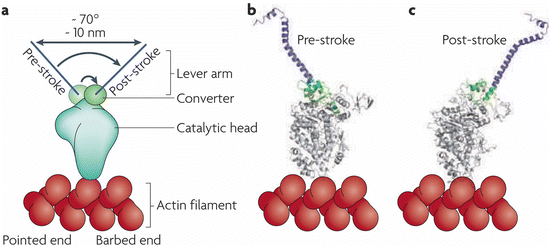
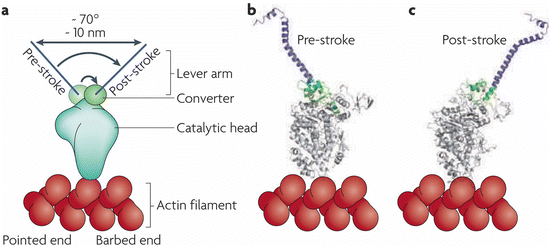
Fig. 6.6
The swinging lever arm mechanism. (a) Schematic representation of the myosin II head and its pre- and post-stroke orientations. (b, c) Corresponding crystal structures illustrating the 70° rotation of the converter and its amplification by the lever arm (from Spudich Nat. Rev. Mol. Cell Biol. 11: 128 © 2010 Reprinted by permission from Macmillan Publishers Ltd)
There are many as yet unanswered questions and unresolved mysteries. One mystery is: where does the energy to drive the dynein, kinesin, and myosin motors come from? Some of the axons that have to be traversed are as long as a meter. Typical step lengths are about 8 nm, and each step requires the hydrolysis of an ATP molecule. The canonical answer is that the needed energy is supplied by mitochondria that are distributed uniformly throughout the axons and dendrites. However, mitochondria are not distributed uniformly but rather are concentrated in synaptic terminals and other high use areas and less so in other regions. So, in those regions devoid of mitochondria, where does the energy come from? A possible answer, and one that is certainly satisfying, is that the motors carry their own energy generators. Specifically, they carry the glycolytic enzyme GAPDH along with them and continuously generate ATP by breaking down sugars. Evidence that this is, in fact, what they may do was uncovered recently.
6.4 Complexes and Aggregates of Misfolded Proteins, and Damaged Organelles, Are Removed through Selective Autophagy
6.4.1 Formation of the Phagophore
The formation of an autophagosome that encapsulates misfolded and aggregated proteins, and malfunctioning organelles, in a double membrane is a remarkable process that begins with initiation and nucleation. That step is followed by an elongation phase and then by capture of the appropriate selective substrates. The phagophore is then closed up to form an autophagosome ready for transport to the lysosome to which it fuses to generate the final structure, the autolysosome (Fig. 6.7). A number of complexes have been identified that orchestrate it by bringing together and helping assemble the lipids needed for the membranes, the protein machinery required to identify and capture the correct substrates, and the proteins that interface to the transport motors.
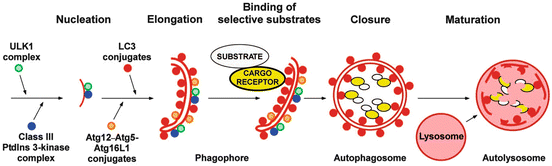
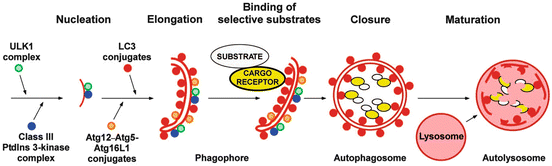
Fig. 6.7
Selective autophagy —steps in the assembly of autophagosomes and autolysosomes (from Johansen Autophagy 7: 279 © 2011 Landes Biosciences reprinted with permission from Taylor & Francis)
Initiation and nucleation: The first complex to act is the Ulk1 complex. This resident complex awaits activating nutrient, energy, and other signals and, once received, begins the recruitment process. It contains in addition to Ulk1 (a serine/threonine kinase) three other proteins—two members of the Atg (autophagy-related) protein family, Atg13 and Atg101, and FIP200 (focal adhesion kinase family interacting protein of 200 kDa). Ulk1 is the mammalian homolog of the yeast Atg1 protein and this complex is often referred to as the Atg1 complex.
Mutual interactions between the Ulk1 and Class III PtdIns 3-kinase complex (Fig. 6.7) occur next leading to nucleation of the phagophore. The latter has two principle members—Beclin 1, a Bcl2 family member, and Vsp34, a Class III phosphoinositide (PtdIns) 3-kinase. Bcl2 proteins are best known as stress sensors and apoptosis signal transducers. The Bcl2 proteins can be grouped into three subfamilies; some, like Bcl-XL and Bcl-2, inhibit apoptosis, while others belonging to the so-called BH3-only subfamily act as stress sensors and when activated promote apoptosis. Beclin 1 is a member of the BH3-only subfamily, but promotes autophagy rather than apoptosis. Under normal growth and nutrient conditions this protein is bound by Bcl-2 or Bcl-XL and remains inactive. Starvation and other stress conditions lead to its dissociation from Bcl-2 thereby enabling it to associate with autophagic proteins and function as a signal integrator. The other member of the complex is Vps34 along with its regulatory subunits Atg14 and Vps15. Vps34 phosphorylates phosphatidylinositol to generate phosphatidylinositol 3-phosphate [PI(3)P] essential for membrane trafficking.
Elongation: The next step, elongation, makes use of a set of operations remarkably similar to the ones used to tag protein for degradation by the proteasome. Like the ubiquitin-protease, a set of E1, E2, and E3 enzymes facilitate the attachment of ubiquitin-like proteins (Ubls) Atg8 and Atg12 to selected substrates. Two sets of conjugates are established. Atg12 is conjugated to its substrate Atg5 to form the Atg12-Atg5-Atg16L1 (E3) complex by the successive actions of Atg7 (E1) and Atg10 (E2) and, similarly, Atg8/LC3 (ubiquitin-like light-chain 3) is conjugated sequentially by Atg7 and Atg3 (E2) to phosphatidylethanolamine (PE) to create lipidated LC3-II complexes.
6.4.2 Substrate Binding and Autophagosome Formation
Upon completion of these steps the LC3-conjugates occupy the inner surface of the nascent phagophore where they provide binding sites for cargo receptors. For many years, disposal via the ubiquitin-proteasome system and disposal through the autophagic-lysosomal pathway were thought to be independent, nonoverlapping processes. That idea was abandoned when selective autophagy emerged as its own process distinct from basal autophagy and in which cargo receptors recognize ubiquitinated substrates and interface them to the LC3s.
One of the key elements (and potential points of failure) in the autophagic-lysosomal pathway is the p62 protein. One of its first properties uncovered in the 1990s was its ability to aggregate proteins for temporary storage. For that reason, its gene was named sequestosome 1 (SQSTM1). Its collection activity is quite selective; this protein binds polyubiquitinated (misfolded) proteins and stores them for later disposal via the autophagic-lysosomal pathway. This protein is a cargo receptor as is NBR1; they bind to ubiquitinated substrates and to the LC3s. The p62-enriched intracellular inclusion s depicted in Fig. 6.8 are referred to as p62 bodies. They contain besides p62 and the misfolded protein substrates, molecular chaperones and ubiquitin E3 ligases. Alfy is a large scaffolding protein found in aggregates of misfolded proteins such as (polyQ) mutant huntingtin and α-synuclein. It functions as an adapter mediating interaction between p62-bound cargo and the Atg12-Atg5-Atg16 complex.
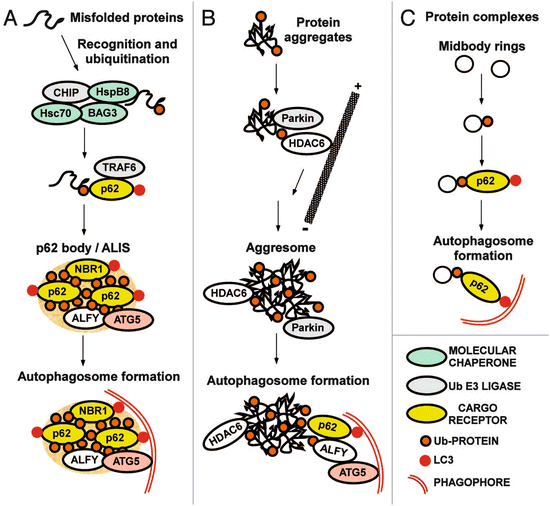
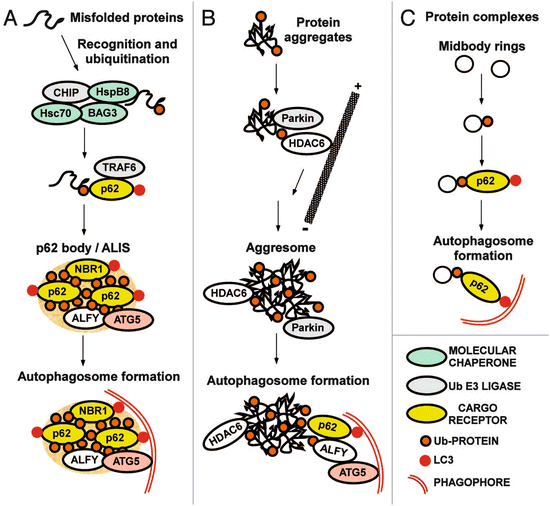
Fig. 6.8
Receptors and adapters involved in selective autophagy . (a) Formation of p62 bodies containing misfolded ubiquitinated proteins. ALIS: aggresome-like induced structure. (b) Formation of aggresomes containing protein aggregates. (c) Putting the pieces together (from Johansen Autophagy 7: 279 © 2011 Landes Biosciences reprinted with permission from Taylor & Francis)
Lastly, autophagosomes are closed, undergo maturation, and are transported by motor protein s along the rail system to where the lysosomes reside in the soma and finally the two compartments fuse. These too are nontrivial operations, and present an impressive number of potential points of failure. However, there are size limitations to entry into the proteasome, and for many large proteins and aggregates, the only available disposal route is by means of autophagy. The disposal of misfolded, aggregated, and ubiquitinated proteins through autophagy is further illustrated in Fig. 6.8.
Aggresomes are inclusion bodies that form in the vicinity of MTOCs/centrosomes. Aggregates are transported to these central locales along microtubule rails by minus-end directed dynein motor protein s . These IBs are characterized by a cage of intermediate filament (IF) proteins, most notably, vimentin. Aggresomes are dynamic entities; they are situated near and recruit components of the ubiquitin-proteasome system and molecular chaperones to deal with the misfolded proteins. The histone deacetylase-6 (HDAC6) protein has a key role in the pathway leading from aggregates to aggresomes to autophagic clearance in lysosomes. This protein is a microtubule-associated protein that regulates microtubule acetylation and the recruitment of dynein motor complexes in a p62-dependent manner. It has an ubiquitin-binding motif that enables it to bind mono-and polyubiquitinated proteins. By possessing this set of properties HDAC6 is able to function as an adapter between ubiquitinated proteins and microtubule-associated dynein motor complexes. Parkin, another component of the aggresome, is an E3 ubiquitin ligase.
Lipids and an alternative pathway: One question that naturally arises is: where do the lipids used in autophagosome biogenesis come from? Several answers have been put forth, each with supporting evidence. The vast majority of the answers postulate that the requisite lipids are derived from a pre-existing membrane rather than being synthesized de novo. Evidence in support of several candidate sources has been found. These include: (1) the endoplasmic reticulum; (2) the Golgi apparatus; (3) mitochondria, and (4) the plasma membrane. Most of these pathways utilize Atg5-Atg7 and LC3 but interestingly, an Atg5-Atg7-LC3-independent-pathway has been uncovered, as well. These observations are not mutually exclusive and it seems that several sources of membrane lipids that can be drawn upon when needed to support autophagy.
6.5 Mitochondria Are Dynamical Entities
Mitochondria are dynamic entities. They readily change their morphology, and continually undergo fission and fusion with each other. In fusion, there is an exchange of materials between the fusing organelles. In this diffusive mixing between healthy and damaged mitochondria, the healthy ones can rescue damaged ones thereby reducing mitochondrial stress. Fusion can increase their size while fission can increase their number and reduce their size. Mitochondrial dynamics and transport have emerged is the last few years as a major subject of research into aging and neurodegeneration. To see why this might be so consider the following:
Neurons have large energy demands. Not only do the molecular machine s that maintain protein heath require continual ATP input, but synapses are huge energy consumers, as well. That aspect was pointed out over 50 years ago by Sanford Palay (1918–2002) who discovered huge concentrations of mitochondria in axon terminals. There, they supply the energy needed for endocytosis, exocytosis, vesicle release, and the mobilization of vesicle reserves. The postsynaptic density is highly enriched in signaling molecules, and neurons maintain a high concentration of mitochondria in dendritic spines and postsynaptic terminals.
Neurons have a unique morphology that puts special demands on the transport system. An adequate energy supply must be maintained over large distances, especially in axons, to the points where consumption occurs. In order to satisfy this need, there is a continual trafficking and recycling of mitochondria from soma to synaptic terminals and back again to the soma for disposal.
Mitochondria are sensitive to buildups in intracellular stress. In response, these organelles may decrease ATP synthesis, increase reactive oxygen species (ROS) production, fail to properly handle Ca2+, and release apoptosis- and necrosis-promoting factors. Declining ATP generation by the electron transport chain and increased oxidative damage correlate with chronological aging .
Mitochondria, like the cell at large, contain a complete multilayer quality control system consisting of an ensemble of mitochondrial chaperones and a damaged-mitochondria transport and macroautophagic disposal network, termed mitophagy . The latter prevents untimely release of death-promoting factors from damaged mitochondria and maintains a proper balance between metabolic adjustments, clearance, recovery, and cell death.
At some point, repair of damaged mitochondria through fusion with healthy ones may fail. Those damaged mitochondria are then removed by the abovementioned mitophagy . Fission may trigger mitophagy by testing daughter membrane potential for functionality. If they fail this stress test they are isolated from the fusion machinery to prevent their contaminating the healthy mitochondrial network, and are shipped via the mitophagy for lysosomal disposal.
6.6 Endoplasmic Reticulum-Associated Degradation and the Unfolded Protein Response
6.6.1 ERAD
Endoplasmic reticulum-associated degradation requires the participation of not only ER-resident chaperones but also E1, E2, and E3 ubiquitin ligases and a variety of recognition, solubility, and bridging factors. In brief, there are four main steps in ERAD. These are:
Recognition in which the misfolded protein is recognized and tagged.
Ubiquitination in which the tagged protein acquires a chain of four or more ubiquitin molecules.
Retranslocation in which the ubiquitinated protein is unfolded and extracted from the ER.
Degradation in which the protein is conveyed to the 26S proteasome for degradation.
One of the most prominent participants in ERAD is BiP, an ER-resident member of the Hsp70 family of molecular chaperones. This protein, like other family members, recognizes exposed hydrophobic patches on the misfolded proteins. Another important participant is p97. The p97 protein is also known as valosin-containing protein (VCP) while in yeast it is referred to as Cdc48. This abundant and versatile protein belongs to the AAA-ATPase family of heptameric ring-shaped proteins discussed in the last chapter. This arrangement enables the enzyme to use the energy of ATP hydrolysis to unfold and extract proteins from membranes, unwind DNA, and disassemble proteins complexes. In addition, p97 protein complexes escort extracted proteins to 26S proteasome for degradation.
6.6.2 The Unfolded Protein Response (UPR)
As noted at the beginning of this chapter, some cell types—neurons, endocrine, and immune cells, in particular—secrete large numbers of proteins. A prominent example is the pancreatic beta cell that secretes insulin. Such proteins utilize disulfide bond formation and glycosylation, and these and other finishing or maturation operations are carried out in the ER’s membranous network. Failure the carry out these modifications adequately because of a buildup in unfolded, misfolded, and/or unfinished proteins is termed ER stress . In order to avoid this condition, cells sense buildups in malformed proteins and make programmatic adjustments intended to return the ER to normal operating conditions. If these protective responses, including autophagy, all fail, that is, if the stresses become chronic, the cells undergo apoptosis.
In order to restore the ER to normal operations signals are sent to both the transcription and translation machinery. These actions not only stimulate increases in the production of chaperones to deal with an increased load, but also slow protein synthesis thereby reducing the growing accumulation of unfolded proteins in the ER. In more detail: (1) Signals are sent that increase expression of genes that remove un- and misfolded proteins either to the UPS or by means of autophagy. (2) Other signals increase the expression of genes that alleviate the stresses (e.g., those encoding chaperones and antioxidants). (3) Still other signals tune down the translational machinery thereby reducing the folding burden placed on the organelle, and lastly, (4) if the stresses are severe and persistent so that protein homeostasis cannot be restored, signals trigger apoptosis leading to destruction of the overburdened cell.
The abovementioned recovery measures are orchestrated beautifully by the three sensors—inositol requiring enzyme 1 (IRE1), PKR-like ER kinase (PERK), and activating transcription factor 6 (ATF6). In the absence of ER stress es, these proteins are inactive residents of the ER membrane. Once they sense excessive stresses, either directly or indirectly, they coordinately mediate the unfolded protein response . The signaling pathways activated by these sensors ER are illustrated in Fig. 6.9. A brief summary of what each one does is, as follows:
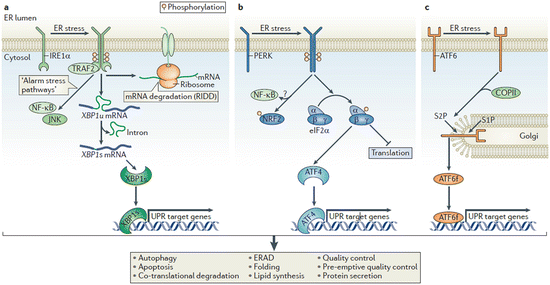
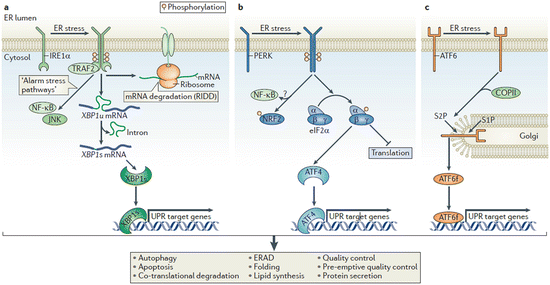
Fig. 6.9
The unfolded protein response . (a) The IRE1 pathway. (b) The PERK pathway. (c) The ATF6 pathway. See text for details (from Hetz Nat. Rev. Mol. Cell Biol. 13: 89 © 2012 Reprinted by permission from Macmillan Publishers Ltd)
IRE1: This protein kinase and endoribonuclease is activated either directly or indirectly by the stressors, in the former case through direct binding and in the latter case through release and relocation of a blocking chaperone such as BiP. In response to these activating events, IRE1 undergoes dimerization and autophosphorylation that creates docking sites. These sites then recruit signaling proteins and initiate multiple downstream actions. These include reduction of the protein folding burden through mRNA degradation and splicing/activation of XBP-1s (X-box protein 1 splice factor), a transcription factor that upregulates chaperones, lipid synthesis, ERAD proteins, and factors needed for autophagy.
PERK: Upon activation by stressors this protein kinase, like IRE1, dimerizes and autophosphorylates. It subsequently phosphorylates eukaryotic translation initiation factor eIF2α thus reducing the protein burden by tuning down most protein synthesis while activating ATF4, a transcription factor that upregulates expression of chaperones, redox enzymes, apoptosis-promoting transcription factors such as CHOP (C/EBP homology protein), and factors required for autophagy.
ATF6: This sensor contains a transcription factor cytosolic domain. Under normal, unstressed conditions it resides in the ER. Stressors induce its translocation to the Golgi apparatus where it is processed by S1P and S2P (site 1 and site 2 proteases) resulting in release of a transcription-competent fragment ATF6f. This transcription factor upregulates ER chaperones that assist in folding such as BiP and Grp94, a Hsp90 family member, along with ERAD components and XBP1s.
Cells take multiple actions to alleviate ER stress . In response, they reroute nascent secretory and membrane proteins from the ER translocon to the cytosol where they are degraded. (The ER translocon is a multiprotein complex built upon the Sec61 channel protein that transports polypeptides into the ER lumen and integrates them into membranes.) This protective process, referred to as co-translocational degradation , is a pre-emptive form of protein quality control that reduces the ER burden. Proteins that require finishing in the ER contain hydrophobic localization signals recognized by the translocon. Mislocalization of these polypeptides to the cytosol on the order of 10–20 % can occur as a result of mutations in their targeting sequences. Aggregation-prone mislocalized proteins are handled by a pre-insertional protein quality control pathway that recognizes and directs them to the proteasome for degradation. Lastly, protein quality control is carried out independently at the inner nuclear membrane (INM). [The outer nuclear membrane is contiguous with the ER.] An ubiquitin ligase complex called Asi (aminoacid signaling independent) has been found in yeast to mediates degradation of INM mislocalized membrane proteins.
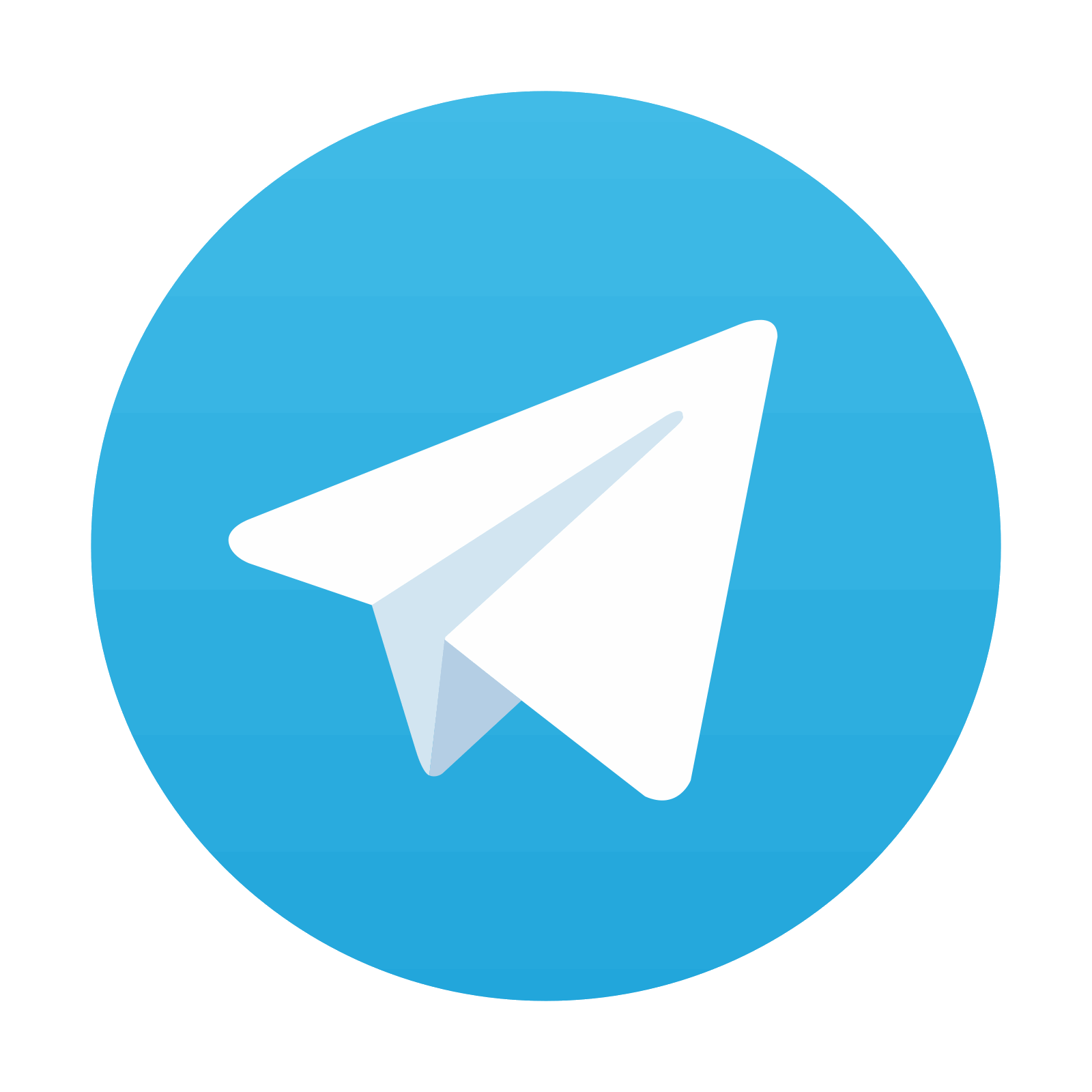
Stay updated, free articles. Join our Telegram channel
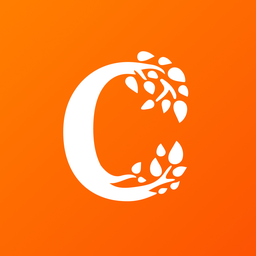
Full access? Get Clinical Tree
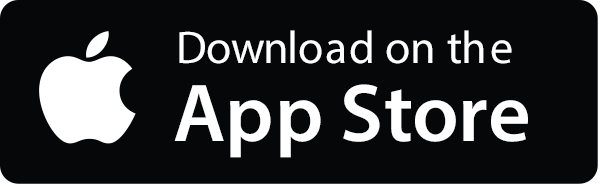
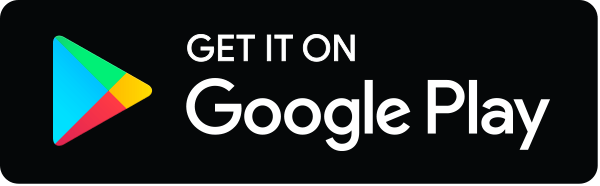