Radiation Risks and Safety
Key Points
Radiation safety lies in the cultivation of good habits and consistent observation of commonsense precautions.
The risk from radiation exposure during neurointerventional procedures is probably small, but it is worth noting that trends in regulatory limits for occupational exposure are becoming more stringent.
Why this Chapter is Important
Since earlier editions of this book, two events have come to pass that have greatly enhanced the importance of this particular chapter on radiation exposure and risk. Firstly, flat detector (FD) technology has ushered in a minor revolution in the capabilities of digital angiography and fluoroscopy. Physicians have at their controls many more operator-determined variables in the modulation of beam intensity and of factors governing patient radiation dose than was possible on older image intensifiers. This renders obsolete both the older technology and the literature associated with it, and places greater responsibility in the hands of the physician for dose reduction. Secondly, the proliferation of CT technology as the primary medical screening tool for virtually all medical disorders has resulted in a quantifiable increase in the average calculated annual radiation dose among the American population, and a critical scientific and journalistic reaction to this has taken hold. A substantial body of jeremiad literature in the popular and medical press now exists expressing qualms on the potential long-term impact of what might be termed this benevolent abundance or wanton profligacy, depending on one’s point of view. Alarming estimates for rates of cancer in the future being induced by current use of diagnostic radiation have been published in establishment journals, and it behooves us to pay attention (1). So far, the focus of concern by regulatory bodies has been on CT angiography and CT perfusion, but the likelihood is that the future will bring similar scrutiny to the neuroendovascular world.
There are several aspects of this problem to consider:
The field of radiation physics and quantification of dose in the diagnostic range is not well understood. Studies show that fewer than 50% of radiologists have an intuitive grasp of the magnitude of dose involved with a chest x-ray, for instance, while a great majority of nonradiologists have difficulties identifying which imaging studies involve ionizing radiation or not (2).
Radiation dose to a patient is difficult to measure directly during a procedure, still more so when the technology involves a biplane room shifting location on the body during the procedure. Therefore we are left with indirect estimates of exposure and dose, built upon measurements of machine output and generic assumptions about factors such as body habitus and table height.
The chain of certainty becomes still more attenuated when one attempts to derive a conclusion on the risk extrapolated from uncertain estimates of dose. These data are, perforce, projected into regression models of risk based on epidemiologic data involving dose rates and populations very different from the individual patient in question.
The following chapter can be summarized, with the greatest parsing of language, by saying that at this time the evidence suggests that the radiation risk associated with neuroendovascular procedures is likely small.
Historical Studies of the Effects of Radiation
An extensive body of epidemiologic and other evidence demonstrates that radiation exposures far in excess of those used for diagnostic procedures are associated with an increased incidence of delayed malignancy. Other delayed side effects of radiation include growth retardation, cognitive impairment, lenticular opacities, premature atherosclerotic vascular disease, and mental retardation after in utero exposure. These data emerge from studies of populations intentionally or inadvertently exposed to significant doses of radiation. These studies include the following:
Patients with ankylosing spondylitis treated with x-irradiation
Patients exposed to Thorotrast
Children with thymic enlargement or tinea capitis who were treated with high doses of local radiation
Adults who were treated with high doses of local radiation to the scalp in infancy for cutaneous hemangiomas
Uranium miners
Radium dial painters
Military personnel exposed during weapons testing maneuvers
Survivors of the Hiroshima and Nagasaki bombings
Victims of the Chernobyl disaster
From studies of exposed populations, data have been collected to give an estimate of the relationship between high radiation doses and biologic effect, with particular emphasis on carcinogenesis. Various regressions of the dose–risk curve down to radiation levels relevant to diagnostic and interventional neuroangiography can be performed on the basis of certain assumptions. The gravity of the conclusions arising from these extrapolations depends on the assumptions made about the lower end of this curve; some assumptions about the curve suggest a higher risk than do others.
To place the nature of risk from radiation in an understandable context, one must first consider that the number of known fatal malignancies following gamma or x-ray irradiation is small compared with more common fatal diseases. This does not trivialize the serious risks from radiation exposure, but places the risk in a relative context.
Concrete comparisons are probably the best medium to demonstrate that, having been exposed to diagnostic or interventional levels of radiation, a radiation-related malignancy is a highly unlikely prospect. Intuitive bias would probably lead most to believe that among atomic bomb survivors, epidemiologic studies should show a massive number of radiation-related malignancies. In fact, although the relative risks of malignancy are high, the absolute numbers are surprisingly low. It is estimated that an exposure of 1 Gy increases the risk of leukemia by a factor of 5.2 (3). To present the risk in a less alarming manner, one might point out that the average exposure of Japanese survivors has been estimated at 0.25 Gy. Of the Japanese survivors enrolled in the Life Span Study (i.e., approximately one-third of the total number of survivors), 37,800 had died by 1990. When one takes expected rates of cancer into account in a similar nonexposed population, the number of excess cancer deaths related to radiation within this group is approximately 430 (4,5,6). The number of radiation-related leukemia cases in the same study had reached 290 by 1987 (7). Stated otherwise, approximately 59% of those survivors who died from leukemia and 8% of the survivors who died from other malignancies did so from a radiation-related cancer (3). Some of these victims received whole body doses of up to 5 Gy (500 rad). Recent data on atomic bomb survivors indicate that a detectable risk of leukemia or lymphoma can be found in groups with exposures as low as 0.5 Gy (50 rad) (8). Although the retrospective dosimetry estimates of the gamma and neutron fluxes involved have been revised periodically, doses involved in these studies, even those revised downward, are not remotely approached by diagnostic neuroangiographic procedures.
Similarly, the absolute numbers of cases of radiation-related leukemia and other malignancies are very small in follow-up studies of other exposed populations. All of the studies that attempt to demonstrate a carcinogenic effect from whole body doses of under 0.05 Gy are of a design and size that make them vulnerable to statistical inconsistencies or methodologic oversights (4,9). Studies of human subjects in this dose range with positive findings have, on occasion, demonstrated an increased risk of malignancy in small populations. For instance, a follow-up study in 1983 (10) of the 1957 “Smoky” bomb test site in Nevada demonstrated an almost four times increased incidence of some forms of leukemia in military personnel exposed to an average of 10 mGy (1 rad) whole body dose. There was no evidence of increased mortality from any other form of cancer. With reference to leukemia, this positive result translates to an extreme risk at variance with the relatively lower observed rates of leukemia in Japanese atomic bomb survivor studies or with risks associated with background radiation (9). Caldwell et al. (10) concluded that “this one positive finding may be either attributable to chance or the result of an unknown combination of factors … this conclusion cannot be generalized.” However, the Nevada test site or other similar events have entered media folklore as evidence of the severe risks of radiation exposure (11).
Epidemiologic studies of atomic bomb survivors and other groups exposed to high levels of radiation (>50 rad) have demonstrated a clear relationship between exposure and the subsequent risk of malignancy, particularly leukemia (excluding CLL) and carcinoma of the lung, ovary, thyroid, stomach, and breast (4). Exposure levels below this range, which would be of interest in evaluating possible risks to patients and medical personnel from neuroangiographic procedures, cannot be studied directly because of methodologic difficulties and because of the enormous number of subjects that such a study would require.
Dose–Response Curve
Using data from populations with known or estimated high-dose exposures, an estimate of risk for low exposures can be performed by a backward regression of the relationship between exposure and outcome. However, this can only be done using assumptions about the lower end of the graph. An extrapolation of the dose–risk curve for radiation exposure below the 100-rad range can be performed in a linear, quadratic, or linear–quadratic manner (Fig. 6-1).
With a linear curve, the risk of malignancy is directly proportional to the exposure. At the lower end of the curve, the number of radiation-related tumors is obscured by the incidence of spontaneous malignancies in a given population. A quadratic curve assumes that the risk is proportional to the square of the dose and gives a curve that increases steeply with increasing doses.
Alternatively, a linear–quadratic curve, in which a linear curve at lower doses gives way to a quadratic curve at higher doses, perhaps in part related to DNA density in mammalian tissue, appears to be a more likely model based on animal studies and on data from atomic bomb survivors. Solid
tumors and leukemia have demonstrated different dose–response curves. Furthermore, a different temporal sequence is seen for various tumors after radiation. The excess incidence of leukemia becomes apparent after 2 years and peaks at approximately 6 years, whereas the incidence of solid tumors may not peak until 15 to 20 years after exposure. A linear–quadratic curve is now most favored for describing the likely risk of most forms of carcinogenesis after radiation exposure. The curve inflection point, where it makes a transition from linear to quadratic in form, could be determined by the density of DNA in the genome (12). This means that for mammalian tissue, the curve changes from linear to quadratic at exposures of approximately 127 rad. Below this level, much of the evidence is either disputed or conjectural, and the measure of risk from an exposure of 10 rad or less is still unknown. For instance, in follow-up studies of patients exposed in infancy to radiation for “thymic enlargement”—another historic fiasco, this time visited upon an unsuspecting public in a stalwart effort to stamp out those two scourges of public health, status lymphaticus and laryngospasm in the setting of normal thymic size variation—the dose–response curve for adult onset of thyroid carcinoma demonstrated a linear regression line (13) even at fairly low doses of thyroid tissue exposure less than 0.3 Gy.
tumors and leukemia have demonstrated different dose–response curves. Furthermore, a different temporal sequence is seen for various tumors after radiation. The excess incidence of leukemia becomes apparent after 2 years and peaks at approximately 6 years, whereas the incidence of solid tumors may not peak until 15 to 20 years after exposure. A linear–quadratic curve is now most favored for describing the likely risk of most forms of carcinogenesis after radiation exposure. The curve inflection point, where it makes a transition from linear to quadratic in form, could be determined by the density of DNA in the genome (12). This means that for mammalian tissue, the curve changes from linear to quadratic at exposures of approximately 127 rad. Below this level, much of the evidence is either disputed or conjectural, and the measure of risk from an exposure of 10 rad or less is still unknown. For instance, in follow-up studies of patients exposed in infancy to radiation for “thymic enlargement”—another historic fiasco, this time visited upon an unsuspecting public in a stalwart effort to stamp out those two scourges of public health, status lymphaticus and laryngospasm in the setting of normal thymic size variation—the dose–response curve for adult onset of thyroid carcinoma demonstrated a linear regression line (13) even at fairly low doses of thyroid tissue exposure less than 0.3 Gy.
So if the bulk of evidence suggests that low-dose radiation risk is a nonstory, wherefore arises the current climate of apprehension?
One of the pivotal papers drawing attention to the altered landscape of radiation use by Brenner and Hall in 2007 (1) made a simple and clear argument that current patterns of CT use translate into a risk of radiation-induced cancer accounting for 1.5% to 2% of all cancers, up from a baseline of 0.4%. In this paper a linear regression model was assumed on the basis of the analyses of atom bomb survivors and a study of 400,000 nuclear industry workers who were exposed to an average dose of approximately 20 mSv (approximately in the range of a couple of CTA scans (14) and in whom an increased risk of cancer was identified. The paper by Brenner and Hall was criticized by several authors for using a linear regression model, for the paucity of hard evidence of risk of carcinogenesis at doses less than 50 mSv, and for not taking into account the putative phenomenon of hormesis. Hormesis is a term to suggest that in response to chronic low levels of radiation, an upregulation occurs in the body’s capacity to deal with free radicals (15). Authors who favor this term invoke it as an argument for a level of impunity to radiation injury, which might, one might facetiously quip, be analogous to saying that one becomes immune to the risk of melanoma by cultivating a deeply bronzed suntan. Evidence suggests that interventional cardiologists as compared with noninterventional cardiologists, for instance, demonstrate increased prevalence of somatic mutations and alterations of DNA repair genes, and while this may be offset by the presence of other markers indicating an increased relative capacity to deal with hydroxyl radicals, they were probably healthier when they were younger before they became cardiologists (16,17,18,19,20,21). Furthermore, the Brenner and Hall paper (1,22) stressed the heightened vulnerability of pediatric patients to the effects of radiation exposure and the fact that for a given examination, due to the thinner torso and head size of children, local organ radiation doses in children are substantially higher than in adults.
X-Ray Physics Review
The realm of radiation exposure and its dangers lie outside of the domain of human experience. For instance, it is difficult intuitively to understand that the energy of a cup of warm water if delivered in the form of high-energy radiation over a brief period of time would be highly lethal to one or more adult humans. Furthermore, the units used to measure and express notions of exposure are derivatives of other derivatives and thus confusing. Therefore, a brief review is necessary of the fundamental principles of radiobiology as a preamble to methods and meaning of radiation exposure.
The adverse effects of x-rays on tissue are related to a transfer of energy to the tissue by indirect ionization. With x-rays in the diagnostic range, the two processes involved, which result in ionization, are the photoelectric effect and the Compton effect. Approximately 5% of x-rays in the diagnostic range undergo coherent scattering. This third process is not important for transfer of energy because only a change of direction of the x-ray photon takes place, without a change in energy and without ionization. Coherent scattering contributes a little to image fogging.
In the photoelectric process, an incident photon interacts with and loses all of its energy to a K, L, or M shell electron, which is then ejected from its orbit. The ejected electron becomes a photoelectron. The ejected electron is replaced in its shell by an electron from an outer orbit, which releases characteristic radiation as it moves to the inner, more tightly bound position. The probability of a photoelectric interaction between a photon and an atom is proportional to the third power of the atomic number of the atom. A photoelectric interaction is also more likely to occur with a lower-energy photon than it is with a higher-energy beam, provided that the photon energy is greater than the binding energy of the electron. The photoelectric effect is therefore likely to occur with photons in the lower-energy range of a diagnostic beam in tissue that contains atoms with a higher atomic number, such as calcium or iodine. Therefore, photoelectric interactions are good for image quality in that they give a high contrast between soft tissue and bone or between soft tissue and iodinated contrast. However, because the photoelectric effect results in all of an incident photon’s energy being deposited in the patient, the absorbed radiation dose to the patient tends to be higher when the photoelectric effect is dominant. Therefore, to reduce patient dose, it is desirable to keep the kV setting as high as possible while maintaining adequate image contrast.
In the Compton effect, the incident photon of the x-ray beam loses part of its energy to an outer electron, which is ejected from its orbit. The ejected electron is referred to as the recoil electron. The x-ray photon continues in a variably deflected direction with a commensurately reduced energy. Compton interactions are responsible for the scatter radiation to operating personnel involved in a fluoroscopy procedure. In the diagnostic range, most of the energy of the incident photon is retained by the photon, and relatively little is transferred to the recoil electron. The practical implication of this is that scatter radiation emanating from a patient is almost as energetic as the photons of the
primary beam. For instance, a 75-keV photon deflected by 90 degrees has an energy of 66 keV; when deflected by 180 degrees, the energy level is still a considerable 58 keV (23). Therefore, the scatter beam, which can be conceptualized as having its center at the skin-entrance site on the patient, must be taken to represent a potentially serious source of radiation to operating personnel (Fig. 6-2).
primary beam. For instance, a 75-keV photon deflected by 90 degrees has an energy of 66 keV; when deflected by 180 degrees, the energy level is still a considerable 58 keV (23). Therefore, the scatter beam, which can be conceptualized as having its center at the skin-entrance site on the patient, must be taken to represent a potentially serious source of radiation to operating personnel (Fig. 6-2).
The ionizing effect of radiation in the diagnostic range is mediated by the photoelectrons and recoil electrons. They result in the formation of free radicals in tissue, which may then interact with other cellular components, particularly with DNA and RNA.
Stochastic and Deterministic Effects of Radiation
The detrimental effects of human radiation exposure are divided into genetic (placing future generations at risk—an effect that has been very difficult to confirm in population studies) and somatic (affecting the exposed individual). Somatic effects can be broadly classified into those related to the local tissue effects of radiation exposure (epilation, skin erythema, necrosis, retrolenticular opacities, etc.) and carcinogenic effects (see Table 6-1).
The confirmed somatic carcinogenic side effects and the putative genetic effects of radiation probably occur through common pathways involving free radical damage to DNA. The risks of these effects are sometimes termed stochastic,
that is, they are best described in terms of probability. Although they are more likely to happen with increasingly higher exposures, the severity of the effect, if it happens, is not dose related. Stochastic effects are considered to be possible at all levels of exposure, that is, they do not have a threshold level. In other words, a radiation-related malignancy is more likely to occur after a higher level of exposure but may occur after a low exposure as well. The net effect to the victim is the same in either instance. A diagnosis of leukemia whether one ascribes it to a Gray or a centiGray of exposure is still leukemia.
that is, they are best described in terms of probability. Although they are more likely to happen with increasingly higher exposures, the severity of the effect, if it happens, is not dose related. Stochastic effects are considered to be possible at all levels of exposure, that is, they do not have a threshold level. In other words, a radiation-related malignancy is more likely to occur after a higher level of exposure but may occur after a low exposure as well. The net effect to the victim is the same in either instance. A diagnosis of leukemia whether one ascribes it to a Gray or a centiGray of exposure is still leukemia.
Table 6-1 Somatic Deterministic Effects of Radiation (24) Skin Effects After Single-fraction Irradiation | ||||||||||||||||||||||||||||||||||||||||||||||||||||
---|---|---|---|---|---|---|---|---|---|---|---|---|---|---|---|---|---|---|---|---|---|---|---|---|---|---|---|---|---|---|---|---|---|---|---|---|---|---|---|---|---|---|---|---|---|---|---|---|---|---|---|---|
|
Nonstochastic effects, sometimes called deterministic, are dose related and affect the exposed tissue. They involve acute or chronic complications such as epilation, ulceration, fibrosis, or lenticular opacities. Usually, they are not seen below a certain critical or threshold level of exposure.
The genetic effects of radiation exposure are probably less significant than the somatic effects. Radiation effects on the genetic material of circulating lymphocytes can be detected with exposures as low as 0.1 Sv. Such changes have been used following the Chernobyl disaster to estimate exposures retrospectively in rescue workers. However, a detectable genetic effect of radiation exposure in subsequent generations after the atomic bombings at Hiroshima and Nagasaki has been elusive. These effects are thought to be small enough to be obscured by the background noise of spontaneous mutational phenomena (4,5,25,26).
Tumor initiation after radiation exposure is the focus of most concern in evaluating the risks of radiation and is probably due to DNA point mutations, base-pair deletions, or translocations. It is not known what factors may place a particular individual at risk for such critical events, but it is known that children are more vulnerable to this effect, as are patients with certain genetic disorders. For instance, patients with some genetic disorders such as xeroderma pigmentosum and ataxia-telangiectasia are more sensitive to the carcinogenic effects of radiation than other members of the general population. Children exposed to fallout contamination after nuclear accidents are more at risk than adults for malignancies such as leukemia and thyroid neoplasia. Children treated with radiation therapy for retinoblastoma also appear to have a higher susceptibility to radiation-induced tumors than do other children or adults treated similarly for other types of tumors.
Promotional effects on tumor genesis may also be important, and these may be independent of radiation exposure. Tumor promotion may involve such factors as the hormonal or dietary status of the patient. For instance, breast tumors after fluoroscopic exposure of the chest in female children are not seen until maturity, indicating that the initiated cell(s) may remain latent until triggered by other stimuli (27,28,29).
Exposure Quantification and Radiation “Dose”
Quantification of radiation takes into account a number of factors, including the intensity of the source, the nature and energy of the source, and the area or particular organs of the patient exposed to the source. A lexicon of terms exists to describe the units of measurement for these variable factors. The most meaningful of these units is the “effective dose.” Calculating the effective dose for a radiographic procedure gives a number in standardized units, which allows comparison between different procedures and permits correlation, up to a point, with probability scales from reference data. The following sections explain the somewhat convoluted derivation of the effective dose.
Quantification of Beam Intensity
Beam intensity is quantified by measuring the ionization charge induced by an x-ray beam in a given mass of air. It is a measurement of intensity that is independent of any biologic effects of the beam or of the presence of tissue in the field. Modern angiography suites are equipped with ionization chambers attached to the exit portals of the tubes. They measure the intensity of the exiting beam and give a reading expressed in units of Gray.meter2 (or equivalent). By using this reading, called the dose–area product (DAP) or air kerma area product, and by calculating the attenuation of the beam between the ionization chamber and the patient, estimations of patient exposure (estimated skin dose [ESD]) can be reached.
Quantification of Energy Deposition in Tissue (“Dose”)
The “dose” of radiation to a person or a volume of tissue is a concept that can have different meanings. A purely physical notion of what constitutes a dose of radiation is the quantity of energy transferred to the tissue by the beam. Energy absorbed by tissue from an x-ray beam in the diagnostic range is measured in gray (Gy). A Gy is defined as an absorbed dose causing a deposition of 1 joule of energy in each kilogram of absorbing tissue. A Gy is the same as 100 rad.
Quantification of Biologic Responsiveness to Radiation: Dose Equivalence
Units such as the gray deal with purely physical parameters. To approach a more meaningful concept of “dose,” account must be taken of the biologic effects of these measurable physical quantities. A Gy (100 rad) of exposure may have different effects on the tissue substrate in question depending on the nature of the radiation source. To take this variable response into account, it becomes necessary to devise a quantity to measure the biologic equivalence of specified exposures. This explains the acronymic derivation of the unit devised for this purpose, the rem—“roentgen equivalent man.” The international unit now in use for expressing the biologic equivalence of radiation dose is the sievert (Sv).
Estimates of biologic dose equivalence—the “dose equivalent”—represented by sieverts are calculated by multiplying the physical dose (Gy) by a weighting factor (WR).
The weighting factor (WR) is determined by the type and energy of the radiation source. A relative weighting factor, WR, is assigned to various radiation types to generate a scale, which reflects the biologic effectiveness of exposure to different sources. Alpha particles have an estimated WR of 20, and neutrons 10, while photon sources have a WR of 1. An exposure of 1 Gy from a source with a WR of 1 gives a dose equivalent of 1 sievert.
Partial Body Exposure: Effective Dose Equivalent or Effective Dose (E)
How is risk to the whole person calculated when only part of the body is exposed to the radiation source? It would seem cogent, for instance, that a certain level of partial body irradiation must represent as great a risk of carcinogenesis as would some lower degree of whole body irradiation. Moreover, partial body irradiation involving the thyroid gland is more dangerous than irradiation involving the extremities only.
To generate a calculation based on partial body exposure, the International Commission on Radiological Protection (ICRP) introduced the effective dose equivalent (HE), now termed the effective dose (E), which is also measured in sieverts.
Various tissues are assigned weighting factors depending on their respective sensitivities to radiation. For instance, thyroid tissue is assigned a tissue-weighting factor (WT) of 0.04. An equivalent dose to the thyroid of 8 mSv could then be calculated to 8 mSv × 0.04 = 0.32 mSv effective dose (HE). In other words, an equivalent dose of 8 mSv confined to the thyroid gland represents the same risk to the individual as if the whole body had received a dose of 0.32 mSv. The most recent revision of recommendations and tissue-weighting factors by the ICRP (30) supersedes those from 1990 (31), and overall downgrades very slightly the risk of radiation-induced carcinogenesis. Some tissue-weighting factors have been altered slightly, for instance WT for the gonads was revised from 0.2 to 0.08, while for breast tissue it was upgraded from 0.05 to 0.12 (32).
To calculate the entire risk to an individual from a quantified exposure, the effective doses for all the critical organs involved are summed to give a number, the total effective dose. This number can be compared with known quantities and risk tables. For instance, the effective annual dose from background radiation exposure is approximately 2.5 to 5.0 mSv, depending on altitude and geologic variables. This is a useful number to remember as a reference point for interpreting the effective dose; it gives one a scale of reference to grapple in a meaningful way with doses involved in medical procedures (Table 6-2; 25,26,33,34,35).
Limitations to the Application of Effective Dose Values
From a reading of medical papers concerning radiation dose and risk, one might gain an erroneous sense of certainty in using the effective dose as a common international exchange unit in the calculation of stochastic or even deterministic risk. This is a common and somewhat misleading assumption. The notion of E is best understood as a control measure, calculated with reference to a theoretical hermaphroditic model and useful for comparing one imaging technique or modality with another (36). It has therefore no real relevance to female organs or breast tissue, or indeed any real relevance to any particular patient. Its accuracy for a particular patient with confidence boundaries of 70% to 90% is probably a matter of ±40%, that is, a wide spread of possibilities (37,38). Its application on a population-wide basis is also premised on the characteristics innate to the population from which it was first derived, that is, the Japanese survivors. How applicable are those data when one considers that mortality rates and incidence of breast cancer, stomach cancer, and other malignancies in the Japanese population have changed considerably since 1945?
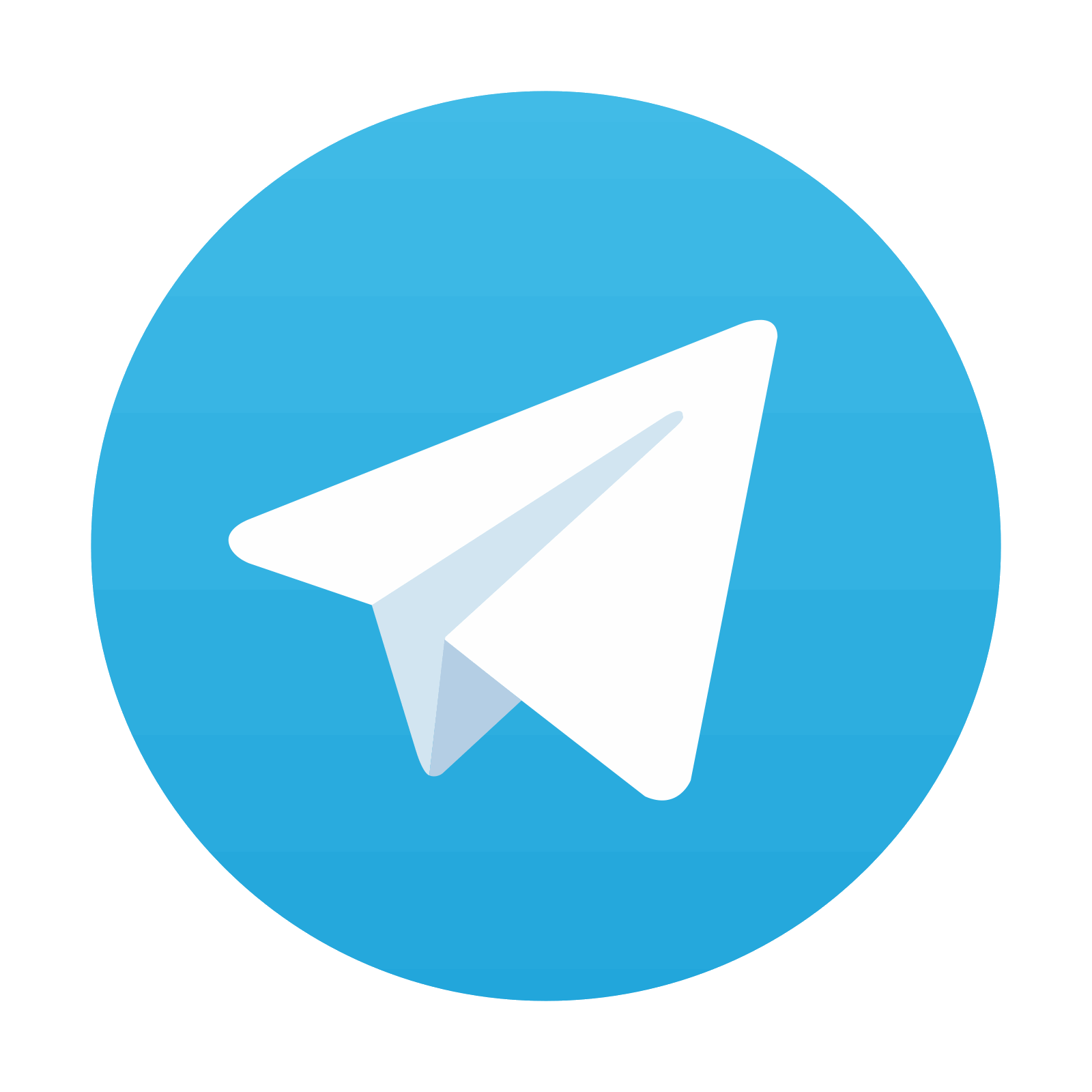
Stay updated, free articles. Join our Telegram channel
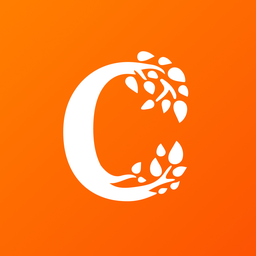
Full access? Get Clinical Tree
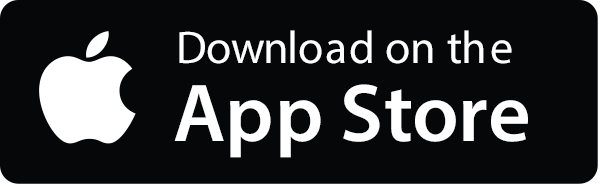
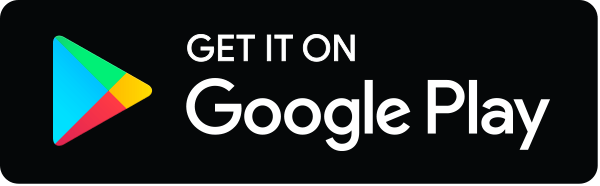