Fig. 19.1
Prone (a) and supine (b) positioning for craniospinal irradiation with personalized thermoplastic mask and vacuum bag immobilizer
19.2.5 Target Volumes, Organs at Risk, and Techniques
The following organs at risk (OARs) will be routinely outlined: eyes, lenses, optic nerves, pituitary gland, optic chiasm, cochlea, brain, supratentorial brain, brainstem, thyroid gland, heart, lungs, breast, liver, kidneys, bowel, bladder, and gonads (if visible). For any of these organs, a dose-volume histogram (DVH) should be constructed for plan analysis. Dose constraints for various OAR are as follows: optic chiasm and optic nerves, 55 Gy [23]; brainstem, 54 Gy [24]; spinal cord, 50.4 Gy [25]; lenses, 8 Gy; and cochlea for sensor neural hearing loss, as low as possible (conservatively ≤35 Gy) [26].
The following target volumes should be observed: craniospinal axis, PF, and tumor bed. Fusion magnetic resonance imaging (MRI) with simulation CT is ideal for planning to determine the extent of presurgical–postsurgical disease. T1 gadolinium-enhanced MRI may be ideal. According to the International Commission on Radiological Units and Measurements (ICRU), specification of volumes and doses is necessary for prescribing, documenting, and reporting therapy [27]. The gross tumor volume (GTV) includes all gross residual tumor and/or tumor bed at the primary site as determined by the preoperative MRI initially defining the tissues involved with disease and the postoperative MRIs identifying residual disease and/or tumor bed. GTV in most cases is a contracted or collapsed tumor bed. Tissue defects resulting from surgical approaches are generally not included as part of GTV.
In the whole brain, the clinical target volume (CTV) should extend frontally to include the entire frontal lobe, the cribriform plate region, and the temporal lobes.
If present, the metastatic deposits should be determined on a planning CT. The field arrangement for boost delivery in these areas must be chosen accurately to provide a high conformity index, avoiding OARs where possible.
The complexity and volume irregularity typical of CSI have led to the development of several new techniques. Relative to the conventional technique, the geometric edge of the cranial shield on the film should extend at least 5 mm below the cribriform plate and 10 mm below the lowest part of the temporal fossa [28]. Often the margin of anterior shielding needs to include the posterior part of the orbit so as to encompass the cranial meninges [17]. The cribriform region should be evaluated with modern techniques like CT or MRI, because conventional treatment planning of the brain area, using lateral radiographs, provides ambiguous information on its localization [29]. If the brain is treated by a pair of parallel opposed fields, the dose should be defined at the midpoint of the central axis.
It is our policy to extend, as much as possible, the lower lip of the cervical spinal volume in the lateral cranial fields avoiding the shoulders. This is advised for three reasons: avoiding most thyroid tissue by shielding this within the cranial volume, minimizing the risk of the junction being close to the primary tumor, and avoiding the exit of the posterior spinal field through the mandible.
The aim of the spinal CTV is to include the entire subarachnoid space with extension along the nerve roots as far as the intervertebral foramina (Fig. 19.2). The width between the intervertebral and the sacral foramina increases no more than 20 mm, as one moves from cranial to caudal regions. The use of a “spade”-shaped field to treat the lumbar-sacral spine is no longer recommended [17]. The lower limit of CTV is the terminal part of the thecal sac, evaluable on a spinal MRI and usually extending inferiorly to at least the lower border of the S2–S3 sacral vertebra. A study on MRI showed that the caudal sac end is at the bottom of S1 in about 17 %, at the bottom of S2 in about 50 %, and at S3 in about 33 % of children [30] (Fig. 19.3a). An additional margin, generally 5–10 mm on CTV, should be added for the planning target volume (PTV), depending on the center’s setup techniques.



Fig. 19.2
The lateral extension of CTV (fuchsia) in the spinal target volume should include the entire subarachnoid space along the posterior spinal ganglia (yellow), located in the intervertebral foramina. Because of a less accurate immobilization of the spinal target compared to the brain target, fixed with mask, a 5–10-mm margin is added for spinal PTV (blue), according to center’s setup policy

Fig. 19.3
Volumetric reconstruction of the craniospinal target (red). The posterior fossa volume is highlighted in blue (a). Axial, sagittal, and coronal MRI reconstruction fuse with simulation CT of pre-operatory GTV (red) and CTV (fuchsia) including the entire posterior fossa and further 5-mm expansion to PTV (blue). Cochlea (green), pituitary gland (yellow), and brainstem (orange) outlined (b) (CT-MRI fusion obtained with Oncentra MasterPlan, Nucletron®, The Netherlands)
The PF is the typical anatomic region in which MB develops. It is delimited superiorly by the tentorium (superior field edge will also be estimated as 10 mm above the midpoint of a line drawn between the foramen magnum and the vertex), posteriorly by the outer table of the skull, frontally by the posterior clinoid (the pituitary should be shielded unless tumor extends to that region), and inferiorly by the outer table of the skull at the foramen magnum with a safety margin of 10 mm, typically at the lower border of C1 (Fig. 19.3b). The brainstem was part of the volume in the entire PF boost irradiation. The reduction of boost volume to the tumor bed instead of the entire PF is under investigation in the current COG-ACNS0331 study for standard-risk patients [31]. The optimal CTV for a reduced-volume posterior fossa boost remains to be defined, although an anatomically confined expansion of 1.5–2 cm around the GTV (preoperative extension based upon the T1 signal changes with and without gadolinium contrast and anatomic shifts or changes after surgery) seems to be reasonable. In standard-risk patients the CTV does not extend to the bony confines of the PF. The brainstem is included in the entire CTV PF boost, but only the portion of brainstem close to the tumor bed is carefully included in the tumor bed boost. Indeed, invasion of the brainstem or cerebellum-pontine peduncle is often the limiting factor for radical resection. The PTV is defined as the CTV with a 3–5-mm margin for both PF boost or tumor bed boost, to account for a day-to-day setup variation and center’s setup techniques. The entire PTV should be covered by at least 95 % of the prescription dose; however, no part of PTV should receive less of 90 % of prescribed dose or more than 110 % of the prescribed dose.
With conventional treatment, the head and the upper cervical spine are treated with parallel-opposed fields. Lateral fields need to be carefully matched over the cervical spinal cord with the spinal field which extends superiorly to form an accurate match with the lower border of the cranial fields. Some centers apply a 5-mm gap between adjacent fields, but this results frequently in a “cold spot.” To avoid over- or underdosage in this region, most centers recommend moving (“feather”) the junction during treatment with a gap of 5–10 mm which does not generate hot-cold spots [17].
We obtain abutting fields, rotating the collimator for the lateral fields to match the divergence of the posterior field and simultaneously the foot of the couch toward the entering lateral fields, with an angle that is a function of the divergent fields (Fig. 19.4a). A second posterior field will be required if the length of the spinal field is excessive and the geometry at this junction differs from that over the cervical spinal cord. To avoid hot spots in the same area, the anatomic junction site needs to be shifted at least 5–10 mm every 9 Gy, once a week. Rather than a classical geometric junction, we prefer a couch rotation with abutting fields in the dorsolumbar region (Fig. 19.4b). This technique avoids delivering a double dose in front of the spinal canal at the level of the junction area, although giving an existing dose to the lower anterior part of the abdomen, a risk for females. When necessary, to reduce the dose inhomogeneity inside the spinal target volume, we use the “field in field” technique (Fig. 19.4c).


Fig. 19.4
Schematic representation of craniospinal irradiation setup for a young child (a) and for a taller patient requiring two posterior fields to cover the entire spinal volume (b). Digitally reconstructed radiographs (DRR) and field geometry reconstruction are shown (c) (Courtesy of Sartor G, Med Physics, CRO Aviano, Italy)
In recent years, it is customary in standard-risk patients to switch from traditional opposite lateral field boost of the entire PF volume to highly conformal techniques like three-dimensional conformal radiation therapy (3DCRT) and intensity-modulated radiation therapy (IMRT).
High conformal techniques in MB have not been confined to the boost but also to addressing CSI volumes. IMRT planning and delivery techniques are increasingly employed. They represent one of the most promising methods of treatment, especially for large and non-regular tumors located close to critical areas. In particular, IMRT may be used as an option for reducing the radiation dose to the cochlea and has also been used to improve homogeneity of spinal RT. Modern techniques to deliver IMRT are HT and volumetric modulated arc therapy (ArcT) (Fig. 19.5).


Fig. 19.5
Midsagittal dose distribution in 4-year-old child affected by standard-risk medulloblastoma. Comparison between conventional craniospinal irradiation (cCSI) (a), arc therapy (ArcT) (b), helical tomotherapy (HT) (c), and intensity modulated proton therapy (IMPT) (d). Conformation to the target increases from cCSI, trough ArcT, and HT, up to IMPT. Exit dose of the prescribed dose to the anterior part of the abdomen is 50–60 % for cCSI, 18 % for ArcT, 10 % for HT, and 0 % for IMPT (cCSI and ArcT plans calculated by Eclipse Varian®, Palo Alto, USA; HT plan calculated by Hi-Art TomoTherapy Inc.®, Madison, USA) (ArcT plan is a courtesy of Chiovati P, Med Physics, CRO Aviano, Italy) (IMPT plan is a courtesy of Widesott L and Amichetti M, ATreP Trento, Italy)
HT is a high conformal technique, allowing RT guided by the images through a system in which a linear accelerator emits a narrow fan beam traveling in a spiral mode [32]. HT is mainly of interest for CSI because of the ability to deliver an IMRT plan, advancing the patient slowly through the gantry, allowing the dose to be sculpted around a complex target, and avoiding issues of beam matching, junctions, multiple isocenter, and beam gaps that are common in conventional CSI techniques [33]. Moreover, with HT technique the children can be placed in supine position, which assures comfort and improves the security during the anesthesia procedures. In our experience in 15 CSI patients younger than 8 years treated with HT, an inspection of DVH reveals excellent conformal quality both for CTV brain and spinal cord with better sparing of OARs close to the target. In comparison with 3DCRT, CSI delivered with HT is able to give a more homogeneous dose and better conformation of the dose to the target, delivering a low-dose bath to the organs around the PTV and slightly increasing the whole body integral dose, which is inherent to the technique [34].
ArcT is instead a dynamic rotational RT using a linear accelerator, in which the gantry speed, multileaf collimator leaf position, and dose rates vary continuously during delivery [33, 35, 36]. To deliver ArcT CSI multiple isocenter is necessary. Generally the first isocenter is located in the brain region; the second isocenter is located in the lumbar region; and in some cases (highest in children or adolescents) the third isocenter is used in the upper thoracic region. For each isocenter a different number of arcs (ranging from 1 to 2) are applied [37]. We generally apply two full arcs (clockwise and counterclockwise) for the brain area and one partial arc for each spinal cord region, with an overlap length of about 3–10 cm for each center. For spinal cord region partial arcs are generated with an avoidance sector in the angle of shoulders and harms for supine positionated patients (e.g., from 50° to 115° and from 245° to 310° arc) (Chiovati P, Med Physics, CRO Aviano, Italy, personal communication) (Fig. 19.6). ArcT has the ability to reduce field junction difficulties that are encountered in conventional treatment by accounting for the overlapping area between arcs during the process of optimization [33, 37]. With this strategy no field matching (potentially leading to hot and/or cold spots) is necessary, and the uniform dose transition task is demanded to the optimizer [37]. This technique has been recently applied in CSI patients and for PF tumors, obtaining similar results than HT both on target and OAR [32, 33, 35, 37] (Fig. 19.5).


Fig. 19.6
Craniospinal irradiation delivered with Arc Therapy (ArcT). Multiple isocenter are necessary. Generally the first isocenter is located in the brain region (two full arcs clockwise and counterclockwise); the second isocenter is located in the dorsolumbar region (one partial arc). For spinal cord region a partial arc is generated with an avoidance sector in the angle of shoulders and harms for supine positionated patients (e.g., from 50° to 115° and from 245° to 310° arc). In this case the length of overlapping region for each field/center is 3.29 cm and the uniform dose transition task is demanded to the optimization process (ArcT plan calculated by Eclipse Varian®, Palo Alto, USA)
19.3 The Rationale of Dose and Volume Reduction in Standard-Risk Patients
Historically the postsurgical treatment for standard-risk patients has been 36 Gy CSI together with a boost of 18–20 Gy to the PF (total dose 54–56 Gy). Under these conditions, the 5-year recurrence-free survival rate was approximately 60 % [38].
Although using such doses has demonstrated a long, recurrence-free survival rate 5 years from diagnosis, there is a spectrum of radiation-related treatment effects. These include hearing loss, endocrine deficiencies, somatic effects, cognitive decline (especially in children younger than 7–8 years), and cerebrovascular damage. Furthermore, the estimated cumulative 10-year rate of secondary neoplasms is 4.2 %, in a population base of 379 standard-risk MB patients treated according to COG trial A9961 with standard RT and adjuvant chemotherapy [39]. Many studies indicate that 2 factors influence the effects of RT: the dose and the age younger than 4 at the time of diagnosis, especially when the 2 are combined. Children who received a lower dose (25 Gy vs. 35 Gy) exhibited less impairment in measures of verbal and visual-spatial intelligence [40], verbal fluency, immediate word list recall, block design, and fine motricity of the dominant hand [41].
Recently, reduced-dose CSI with 23.4 Gy and a boost to the PF (total dose 55.8 Gy) have been used for standard-risk MB (Table 19.1).
Table 19.1
Radiotherapy recommendations
Risk-adapted craniospinal irradiation in medulloblastoma patients | |
---|---|
Standard risk | CSI 23.4 Gy |
PF boost up to 55.8 Gy (reduction of the boost volume to the tumor bed is currently being investigated) | |
Post-RT chemotherapy | |
High risk residual >1.5 cm | CSI 36–39.6 Gy |
PF boost up to 55.8 Gy | |
Combined with chemotherapy | |
Second look surgery | |
High risk M+ | CSI 36–39.6 Gy |
PF boost up to 55.8 Gy | |
Nodular leptomeningeal disease up to 45–50 Gy | |
Combined with intensified chemotherapy (pre- and/or post RT) | |
HART could be considered | |
Infant (<3 years old) standard risk | Chemotherapy alone for selected patients |
In desmoplastic/nodular variant, elimination of RT could be an appropriate strategy | |
RT is delayed as much as possible using chemotherapy | |
RT possibly restricted to the posterior fossa (54 Gy) | |
Infant (<3 years old) high risk | Intensified chemotherapy |
Second look surgery | |
RT is delayed as much as possible using chemotherapy | |
Local RT (54 Gy) and/or age-adapted CSI (18–23.4 Gy) is required in these subgroups | |
Nodular leptomeningeal disease 45 Gy |
The minimal dose of RT needed for disease control is still unknown, but since the 1990s, several authors reported their experience of reduced-dose CSI. In a combined Children’s Cancer Group (CCG, 923) and Pediatric Oncology Group (8631) study, patients 3–21 years of age with standard-risk MB were randomized to receive either standard-dose (36 Gy) or reduced-dose (23.4 Gy) CSI, followed by a PF boost. The protocol was terminated prematurely because of early relapses in the reduced-dose arm. Long-term follow-up confirmed this data, with a 67 % EFS at 5 years for patients treated with standard-dose CSI and 52 % for the reduced dose (p = 0.08) [42].
If, on one hand, this trial failed to formally demonstrate equivalent survival after reduced-dose CSI, on the other hand, adding combination chemotherapy allowed goal achievement. Packer et al. investigated the use of reduced-dose CSI of 23.4 Gy with a PF boost to 55.8 Gy, followed by chemotherapy in carefully selected standard-risk patients. Chemotherapy consisted of 8 cycles of vincristine, lomustine, and cisplatin. Results showed 5-year progression-free survival (PFS) of 79 %. This outcome supported the use of reduced-dose CSI along with adjuvant chemotherapy for patients with standard-risk MB [43].
The evolved standard for MB standard risk now includes postoperative CSI to 23.4 Gy, irradiation of the anatomic PF to 55.8 Gy, and about 11 months of combination chemotherapy [44, 45].
Unfortunately, studies on intellectual outcome of children treated for MB showed significant neurocognitive complications even after 23.4 Gy of CSI, especially in younger children. Mulhern et al. reported on the neuropsychological outcome for 22 children. At a median time of 8 years from diagnosis, patients had a median Full-Scale IQ of 82.9. Children who were younger than 8 years of age at diagnosis and received 23.4 Gy CSI (median Full-Scale IQ = 85 for 5 children) had a median 15 IQ point advantage when compared to children receiving full-dose CSI to 36 Gy (median Full-Scale IQ = 70 for 6 children) [46]. Ris et al. describe the significant decline over time in both intellectual and academic domains in a population of 110 patients (COG A9961) treated with 23.4 Gy CSI plus adjuvant chemotherapy. The decline was greater in children who were younger at diagnosis and had higher initial intelligence test score [47].
Since intellectual outcome with these protocols remains suboptimal, investigators are now considering new ways to further reduce the side effects of RT. The first approach is an attempt to reduce the neuraxis dose to 18 Gy. There are only limited data on the efficacy of this strategy [48], and other studies are ongoing [31]. An effective method is targeting less than the entire PF for the boost phase of therapy. Among others, the St Jude Group explored the role of conformal RT boost in 86 newly diagnosed standard-risk MB subjects. RT began within 28 days of definitive surgery and consisted of CSI (23.4 Gy), conformal to PF (36 Gy), and primary site RT (55.8 Gy). Five-year EFS was 83 %, comparable to historical CSI and PF. The targeting guidelines used in this study resulted in a mean reduction of 13 % in the volume of PF receiving doses >55 Gy compared with conventionally planned RT. The dose reductions to the temporal lobes, cochleae, and hypothalamus were statistically significant. The 5-year cumulative incidence of 4.9 % local failure was comparable to results reported for similar patients treated with 23.4 Gy CSI and adjuvant chemotherapy in which the entire PF was irradiated to 55.8 Gy [49]. These data have been confirmed in a recent study by Paulino et al. in which the PF and tumor boost were delivered with IMRT technique [50]. These studies have been built to reduce the side effect of RT and are based on consideration that local failure in the PF outside the tumor bed is rare as the solitary site of failure [2, 3].
Based on this data, the Children’s Oncology Group is currently undertaking a 4-arm clinical trial for patients between 3 and 21 years of age with standard-risk MB. Children aged 3–7 years are randomized twice: one randomization will determine whether the child receives standard (23.4 Gy) or reduced radiation to the brain and spine (18 Gy) and the second randomization will determine whether they receive a standard RT boost to the entire PF or reduced boost to the tumor bed only (total dose 55.8 Gy). Children of 8 years or older will all receive the standard radiation dose to the brain and spinal cord, but will be randomized to either a standard or reduced-volume “boost” dose. In this study, children receive weekly vincristine during RT and lomustine, vincristine, cisplatin, etoposide, and cyclophosphamide after RT. The final data collection date for primary outcome measure is scheduled in July 2016 [31].
An additional side effect is hearing loss that usually develops within 6–12 months after cochlea irradiation (Fig. 19.7). Although the mechanism remains unproven, it is generally thought to be caused by RT-induced changes in the cochlea or vasculature [51]. Platinum agents play an important role in chemotherapy regimens for MB, but also in inducing ototoxicity. It has been shown to be even more significant when RT and cisplatin chemotherapy are used in combination. Schell et al. predicted that the probability of developing substantial hearing loss with cranial radiation was 40–60 % at cisplatin doses of 270 mg/m2. With doses of 450 mg/m2, the risk increased to 80–100 % [52]. Conventional treatment of the entire PF with 2 opposite fields will irradiate the cochlea fully. Instead, the application of conformal techniques like 3DCRT, IMRT, or PRT to PF [51] or of a reduced-volume PF boost [36, 49] is an attempt to limit the cochlear dose. 3DCRT and IMRT reduce cochlear doses to less than 70 % of the mean target dose [53]. In our experience, HT-IMRT used for PF boost is able to reduce the cochlear area dose to less than 50 %, simultaneously decreasing the higher dose to the temporal lobes and supratentorial brain, although there is a greater spread of medium dose toward the pituitary region (Fig. 19.8).



Fig. 19.7
Axial CT image of 18-month-old girl through the skull base. Cochlea (red), vestibule (blue), and internal auditory canal (green) outlined

Fig. 19.8
Posterior fossa boost and skull base axial dose distribution in 18-year-old female affected by standard-risk medulloblastoma. A dose-volume analysis for the cochlea is not easy reproducible due to its small volume and the limitations with its delineation: so the cochlea volume (blue) has been deliberately enlarged to obtain a better optimization in this area. DVH comparison between conventional RT, using two parallel-opposed lateral fields (a), and helical tomotherapy (HT) (b): reduced cochlea region irradiation to less than 50 % of prescribed dose. Simultaneously HT treatment reduces the higher doses to temporal lobes (red) and supratentorial brain (green), but increases low-intermediate doses (c) (Conventional posterior fossa boost plan calculated by Eclipse Varian®, Palo Alto, USA; HT plan calculated by Hi-Art TomoTherapy Inc.®, Madison, USA)
Finally, investigators worldwide have tested different strategies to reduce treatment-related toxicity using hyperfractionated radiation therapy (HFRT) for both the CSI and boost phases. HFRT is a technique that separates radiation into 2 doses per day, giving a higher overall dose, theoretically increasing the chance of irradiating actively dividing cells without killing normal cells. In HIT-SIOP PNET 4 trial, 340 standard-risk patients were randomly assigned to receive HFRT (36 Gy, 1 Gy/fraction b.i.d. to the craniospinal axis followed by a hyperfractionated boost to the whole PF at a dose of 60 Gy with a further boost to the tumor bed at a dose of 68 Gy) or standard conventional fractionated RT (23.4 Gy to the craniospinal axis and 54 Gy to whole PF, given in 30 daily fractions of 1.8 Gy each). Thus, the HFRT technique aims to improve the therapeutic ratio, either by enhancing the antitumor effect without an increase in later side effects or by maintaining the same level of antitumor effects and reducing later morbidity. In both arms adjuvant chemotherapy started 6 weeks after the end of RT, consisting of 8 cycles of cisplatin, lomustine, and vincristine. The OS and EFS in the HFRT arm were not superior to standard fractionated RT group [54].
19.4 Proton Beam Radiation Therapy
PRT currently represents the most conformal approach for CSI. Protons provide a dose distribution that cannot yet be achieved by the most sophisticated photon beam treatment planning. The major disadvantages of PRT are the restricted access and high cost.
When protons come to the end of their penetration range and stop, they deposit all their radiation dose (Bragg peak). Beyond the peak, no further dose deposition occurs with a clear reduction of unintended irradiation of OARs [58], despite active debate on secondary neutron contamination. Bragg peak can be employed to achieve a high conformation around the target. Indeed charge particles (like protons) have a unique advantage over photons because of the ability to confine the high-dose region to the tumor volume and minimize the dose to the surrounding tissue [59, 60], thus reducing the potential for treatment-related complications.
Neurocognitive damage is generally considered the most devastating sequelae; however, protons cannot reduce this by themselves. PRT increases dose conformation to the PF and subsequently reduces the higher doses to supratentorial brain [61].
Long-term toxicity with emphasis on hearing, endocrine, cardiac, gonadic function, and secondary tumors should be substantially improved secondary to nontarget tissue sparing achieved with protons [62]. Sparing of these OARs may be an additional advantage in assuring long-term, posttreatment morbidity-free survival [63]. With conventional photons, the PF boost delivers a substantial dose to the cochlea, spared with PRT, limiting radiation-induced ototoxicity [61]. The dose to 90 % of the cochlea was reduced from 101.2 % of the prescribed PF boost dose from conventional X-rays to 33.4 and 2.4 % from IMRT and PRT, respectively. Dose to 50 % of the heart volume was reduced from 72.2 % for conventional X-rays to 29.5 % for IMRT and 0.5 % for PRT [64]. With the future development of intensity-modulated proton therapy (IMPT), both the exit and entrance dose could be further reduced with less normal tissue unnecessarily irradiated (Fig. 19.5 ) and integral dose sparing [58], and also the neutron component is decreased compared to passive scattering technique. As more hospital-based proton treatment centers become operational, prospective trials that assess the late toxicity of different radiation techniques are needed to substantiate the expected reduction in long-term side effects with PRT.
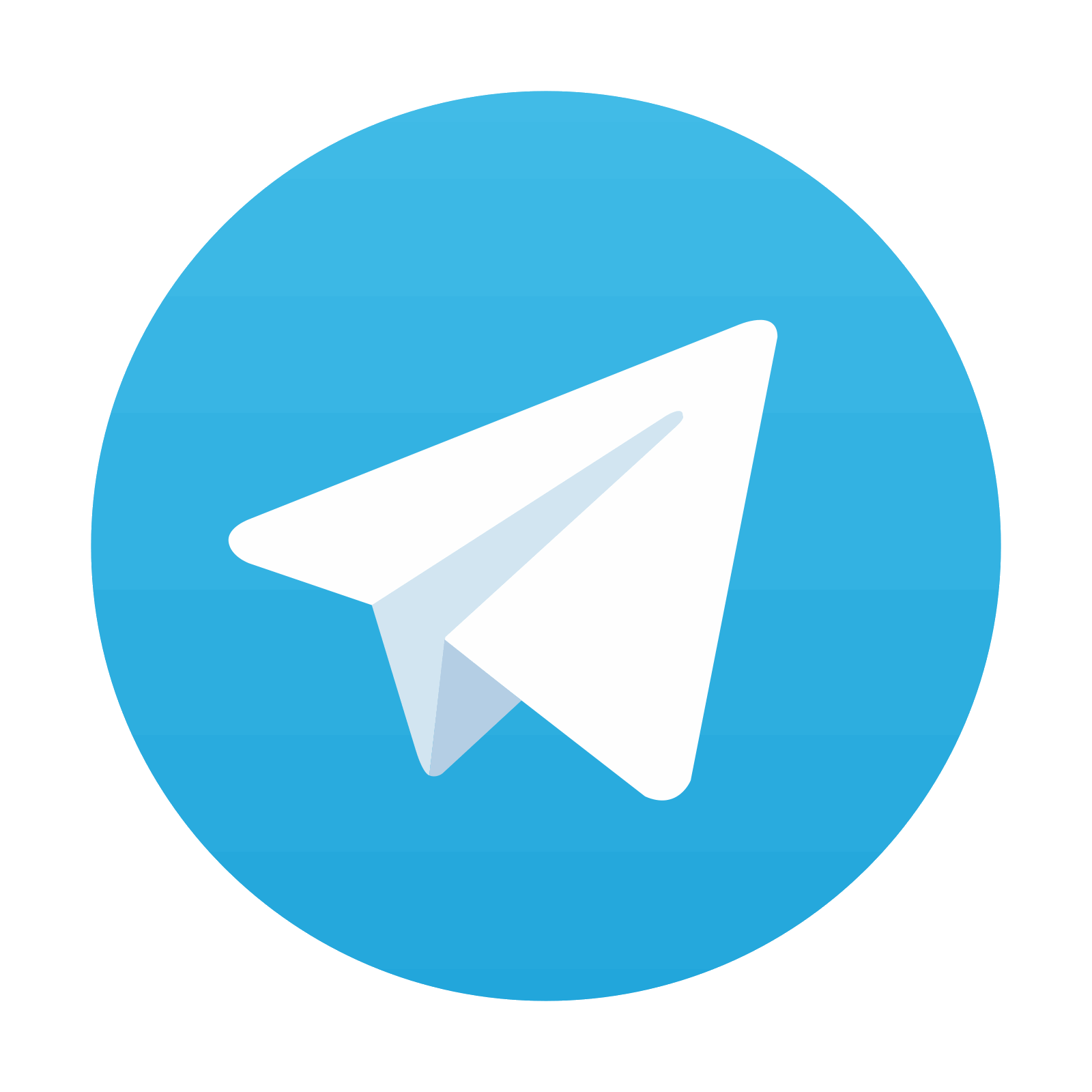
Stay updated, free articles. Join our Telegram channel
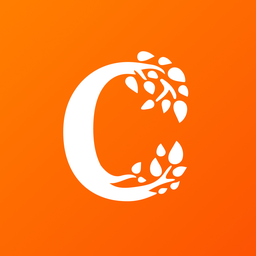
Full access? Get Clinical Tree
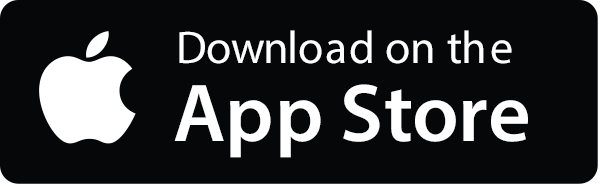
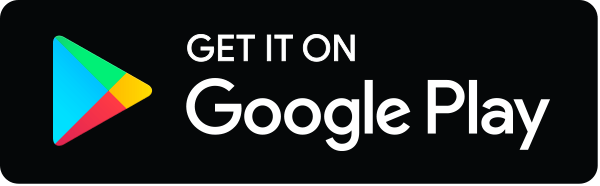