Fig. 36.1
Focal brainstem glioma, sagittal T1 image
Over the same period improvements in neurosurgical techniques and perioperative care have made surgery feasible for all except diffuse intrinsic tumors [1].
The classically quoted incidence of brainstem tumors in children identifies 70–80 % as diffusely infiltrating lesions (primarily arising in the pons) with the remainder identified as one of the focal or benign tumor types. They arise in the pons and cause diffuse enlargement of the brainstem (Fig. 36.2). Extension to the midbrain and medulla and/or exophytic growth is seen in at least two thirds of cases. The histologies are usually fibrillary astrocytomas with a potential for malignant change and have a very poor prognosis. The most common presenting symptoms for the diffusely infiltrating pontine gliomas include diplopia, motor weakness, and difficulty with speech, swallowing, and walking. Neurologic signs include ataxia, cranial nerve palsies (most commonly pontine nerves followed by the medullary nerves), and long tract signs. Only 10 % of the lesions have hydrocephalus at diagnosis. Tumors of the midbrain and medulla may be diffuse or focal, even diffuse tumors show much less infiltration and expansion of the brainstem than seen with the pontine gliomas.


Fig. 36.2
Diffuse pontine glioma, axial T2 image
The duration of symptoms correlates with the type of brainstem glioma. Children with diffusely infiltrating pontine gliomas have a brief history of neurologic symptoms almost appears in weeks and usually less than 6 months. The more focal, less aggressive brainstem tumors are often associated with prolonged symptoms, typically confined to deficits in one or two cranial nerves alone unlike diffusely infiltrating tumors.
MRI is the definitive test for diagnosis and delineation of tumor extent and type. The typical diffusely infiltrating pontine glioma is homogeneous or hypodense on T1 imaging. The tumor expands the pons and infiltrates into other brainstem segments. They are best seen on T2 weighted or FLAIR MRI which should be the imaging modality of choice. There is often little or no enhancement, but inhomogeneous areas of enhancement can be noted [2]. The presence of a ring enhancement is suggestive of high-grade histology. Biopsy of the classic, diffusely infiltrating pontine glioma is generally unnecessary, and there is no clear benefit derived from biopsy when the imaging and clinical picture show a typical diffusely infiltrating tumor. Histological series show equal proportions of low and high grade [3]. There is no consistent correlation between histology and outcome; generally all diffusely infiltrating pontine tumors show poor duration of response to irradiation, and median survival is less than 1 year [4–9]. It is of interest that most such tumors show malignant astrocytoma at autopsy even the ones showing low-grade histology at initial diagnosis.
Brainstem tumors have recently been shown to express ERBB1 with the degree of overexpression is proportional with increasing g grade [10]. These findings suggest that EGFR inhibitors can be worth studying in these kinds of tumors in conjunction with radiation. One of the studies ongoing among the Pediatric Tumor Consortium examines gefitinib and radiation in pediatric patients newly diagnosed with brainstem tumors in a phase I/II manner.
The management of pediatric brainstem gliomas basically depends on the location of the tumor and the specific imaging characteristics of the tumor. Patients with nonenhancing focal tumors in the tectal region who present with only hydrocephalus may do well without any treatment other than CSF diversion. Active intervention including biopsy is reserved for clinical and radiologic progression. Surgery is the treatment of choice for focal tumors at other locations that are surgically accessible (either they extend toward the surface of the brainstem laterally or at the floor of the fourth ventricle) and have imaging characteristics of low-grade histology (uniform bright enhancement with contrast material which correlated with pilocytic histology, the absence of peritumoral hypodensity) as mentioned above. Similar to completely or subtotally resected low-grade gliomas at other sites results can be excellent in a high percentage [3, 11]. Results are less satisfactory for patients with bulky tumors and for patients in the medulla with lower cranial nerve deficits because of high risk of postoperative complication in these cases. For surgically inaccessible focal lesions, conventional radiotherapy should be the standard of care. Looking at the treatment results of the series using conventional radiotherapy in the brainstem tumors, it is reasonable to assume that 50–70 % of focal lesions may be permanently controlled with such treatment [2–4, 9, 12]. Dorsal exophytic lesions are treated with surgery, and ultrasound guidance is used to achieve maximal tumor resection during the procedure. However, as there is no definite interface between tumor and brainstem in most of the cases, there are usually a thin layer of residual tumor cells after the surgery on the floor of the fourth ventricle. Nonetheless there is no standard postoperative radiation therapy for these kinds of tumors as the majority of the children do well following surgery. Radiotherapy should be considered for patients that found to have high-grade lesions or for patients that have low-grade tumors who are found to have progressive disease in the first 1–9 months after the surgery. For patients that recur after this period re-surgery, further surgery should be considered and radiation should be reserved for those who have inoperable disease. According to the literature, the salvage is possible with excellent overall prognosis, especially for dorsal exophytic lesions [11, 13, 14].
Cervicomedullary lesions arise in the upper cervical cord and grow rostrally beyond the foramen magnum, and they are usually low-grade lesions whose axial growth is limited by the pyramidal decussations located at the junction of cervical cord and medulla. The tumor grows posteriorly causing a bulge in the dorsal of medulla toward the fourth ventricle. Surgery is the treatment of choice in these tumors with a gross total resection achieved in 75 % of the cases and subtotal resection in the remaining 25 %. For the typical low-grade lesions, outcomes after surgery are excellent. There is no routine indication for postoperative radiotherapy for these kinds of lesions. Radiation is reserved for surgically inaccessible lesions and high-grade tumors.
Surgery has no role in the management of diffuse intrinsic lesions. Even biopsy is no longer considered as necessary in the context of a typical clinical presentation with the characteristic MRI findings; histology does not affect the outcome of these patients and does not influence the treatment [15, 16]. In conventional radiation, the majority of the patients (75 % and over) with diffuse intrinsic tumors improve clinically. The improvements in signs and symptoms begin after several weeks during and after irradiation. Improvement in MRI has been reported in 30–70 % of children. However, the progression-free interval is short with a median of less than 6 months, and survival is poor with median survival less than 1 year and survival rates at 2 years is less than 20 % [3–5, 7, 12, 17]. Intrinsic focal pontine lesions often must be irradiated at diagnosis because of the neurological signs. With the availability of the precision volume techniques, the risk benefit ratio may favor early intervention in these lesions.
36.1 Technology of Radiotherapeutic Management
36.1.1 3D Conformal Radiotherapy
Powerful x-ray CT simulation and three-dimensional treatment planning systems (3DTPS) have been commercially available since the early 1990s, and three-dimensional conformal radiation therapy (3DCRT) is now firmly in place as the standard of practice. In addition, advances in radiation treatment-delivery technology continue, and medical linear accelerators now come equipped with sophisticated computer-controlled multileaf collimator systems (MLCs) and integrated volumetric imaging systems that provide beam aperture and/or beam-intensity modulation capabilities that allow precise shaping and positioning of the patient’s dose distributions. Conformal treatment plans generally use an increased number of radiation beams that are shaped to conform to the target volume (Figs. 36.3, 36.4, and 36.5). Forward-based 3D planning for conformal therapy typically involves a series of procedures summarized below:




Fig. 36.3
3D conformal radiotherapy with two-field technique

Fig. 36.4
3D conformal radiotherapy with three-field technique

Fig. 36.5
3D conformal radiotherapy with five-field technique
Step 1: Patient positioning and immobilization
Step 2: Image acquisition and input (CT, MRI, or other imaging data)
Step 3: Anatomy definition (contours for organs at risk, target volumes)
Step 4: Dose prescription (for planning target volume and organs at risk)
Step 5: Beam technique (beam arrangements, field shape, beam modifiers, beam weighting)
Step 6: Dose calculations
Step 7: Plan evaluation/improvement (two- and three-dimensional displays, dose volume histograms, and isodose curves)
Step 8: Plan review and documentation
Step 9: Plan implementation and verification
36.1.2 Intensity-Modulated Radiation Therapy
Since its introduction into clinical use, intensity-modulated radiation therapy has generated widespread interest. IMRT optimally assigns nonuniform intensities to tiny subdivisions of beams, which have been called rays or beamlets. The improved dose distributions potentially may lead to improved tumor control and reduced normal tissue toxicity (Fig. 36.6). IMRT requires the settings of the relative intensities of tens of thousands of rays comprising an intensity-modulated treatment plan. This task cannot be accomplished manually and requires the use of specialized computer-aided optimization methods. IMRT dose distributions can be created to conform much more closely to the target volume, particularly for those volumes having complex/concave shapes, and shaped to avoid critical normal tissues in the irradiated volume. This increased conformality results in IMRT treatments being much more sensitive to geometric uncertainties than the two-dimensional or 3DCRT approaches and has spurred the development of treatment machines integrated with advanced volumetric imaging capabilities, which is again pushing the edge of our frontiers in conformal therapy practice from IMRT to what is now referred to as image-guided IMRT or simply image-guided radiation therapy (IGRT). It should be understood that most of concepts and tasks discussed apply equally well as in 3DCRT to IMRT and IGRT, particularly with regard to target volume definition.


Fig. 36.6
Intensity-modulated radiation therapy technique
36.1.3 Stereotactic Radiosurgery and Radiotherapy
Stereotactic radiosurgery and radiotherapy are techniques to administer precisely directed, high-dose irradiation that tightly conforms to an intracranial target to create a desired radiobiologic response while minimizing radiation dose to surrounding normal tissue. These techniques exploit the fact that the radiation tolerance of normal tissue is volume-dependent. With these techniques, the complication risks for any radiation dose delivered are reduced by minimizing or eliminating the margin of normal tissue otherwise included in the radiation treatment volume with conventional radiotherapy techniques. In the case of radiosurgery, all of the irradiation is done in a single session or fraction, while in stereotactic radiotherapy (SRT), more than one fraction of irradiation is administered. The key requirements for stereotactic treatments should be:
1.
Small target/treatment volume: Reduction of the volume of normal and target tissue irradiated to high doses improves tolerance.
2.
Sharply defined target: Can be treated with little or no extra margin of surrounding normal tissue and/or without unintentional under dosage of the target (marginal miss).
3.
Accurate radiation delivery: No margin of normal tissue is needed for setup error and/or reduced chance of underdosing target.
4.
High conformality: Reduces the treatment volume to match the target volume.
5.
Sensitive structures excluded from target: Dose-limiting structures (optic chiasm, spinal cord) should be able to be defined and excluded from the target volume to limit the risk of radiation injury.
Advances in imaging, computers, and treatment planning in the last two decades have led to the development of a variety of different stereotactic radiosurgery/radiotherapy techniques and their wider applications. Successful clinical experience with intracranial radiosurgery for a variety of applications has led to a reexamination of radiobiology and exploration of both fractionated approaches and extracranial applications. Margin reduction with radiosurgery and fractionated stereotactic irradiation techniques makes target definition accuracy more critical.
36.1.4 Volumetric Arc Therapy
Improvements in patient care achieved through image-guided positioning and plan adaptation have resulted in an increase in overall treatment times. IMRT has also increased treatment time by requiring a larger number of beam directions, increased monitor units (MU), and, in the case of tomotherapy, a slice-by-slice delivery. The solution in volumetric arc therapy is a novel aperture-based algorithm for treatment plan optimization where dose is delivered during a single gantry arc of up to 360°. The technique is similar to tomotherapy in that a full 360° of beam directions is available for optimization but is fundamentally different in that the entire dose volume is delivered in a single source rotation. The new technique is referred to as volumetric-modulated arc therapy (VMAT). Multileaf collimator (MLC) leaf motion and number of MU per degree of gantry rotation is restricted during the optimization so that gantry rotation speed, leaf translation speed, and dose rate maxima do not excessively limit the delivery efficiency. During planning, investigators model continuous gantry motion by a coarse sampling of static gantry positions and fluence maps or MLC aperture shapes. The technique is unique in that gantry and MLC position sampling is progressively increased throughout the optimization. Using the full gantry range will theoretically provide increased flexibility in generating highly conformal treatment plans (Fig. 36.7). In practice, the additional flexibility is somewhat negated by the additional constraints placed on the amount of MLC leaf motion between gantry samples. A series of studies are performed that characterize the relationship between gantry and MLC sampling, dose modeling accuracy, and optimization time. The competing benefits of having small and large sample spacing are mutually realized using the progressive sampling technique described here. Preliminary results show that plans generated with VMAT optimization exhibit dose distributions equivalent or superior to static gantry IMRT. Timing studies have shown that the VMAT technique is well suited for online verification and adaptation with delivery times that are reduced to approximately 1.5–3 min for a 200 cGy fraction.


Fig. 36.7
Volumetric arc radiation therapy technique
36.2 Treatment Volume
36.2.1 Definitions of Tumor and Target Volumes
The International Commission on Radiation Units and Measurements (ICRU) first addressed the issue of consistent volume and dose specification in radiation therapy with the publication of ICRU Report 29 in 1978 [18]. In retrospect, ICRU Report 29 recommendations were well suited for the technology of the 1970s and 1980s, that is, using a conventional simulator to generate a planning radiograph for designing beam portals based on bony and soft tissue landmarks for standardized beam arrangement techniques applied to whole classes of comparable patients.
In 1993, the ICRU updated its recommendations for specifying dose/volume in Report 50 and were well suited for conformal therapy [19]. The target volume definition was separated into three distinct volumes: (a) visible tumor, that is, gross tumor volume (GTV); (b) a volume to account for uncertainties in microscopic tumor spread, that is, clinical target volume (CTV); and [3] a volume to account for geometric and other uncertainties, that is, planning target volume (PTV) as illustrated in Fig. 36.8.


Fig. 36.8
ICRU definitions for target volumes
The GTV and CTV are anatomic-clinical concepts that should be defined before a choice of treatment modality and technique is made. Labels or subscripts with the GTV nomenclature can be used to distinguish between primary disease and other areas of macroscopic tumor involvement. Note that even if the GTV has been removed by radical surgery, the volume can be designated as CTV. In specifying the CTV, the physician must not only consider microextensions of the disease near the GTV but also the natural avenues of spread for the particular disease and site. The PTV is defined by specifying the margins that must be added around the CTV to manage the effects of organ, tumor and patient movements, inaccuracies in beam and patient setup, and any other uncertainties. The PTV is a static, geometrical concept used for treatment planning and for specification of dose. Its size and shape depend primarily on that of the GTV/CTV, and the effects caused by internal motions of organs and the tumor, technical aspects of treatment technique (e.g., patient fixation). The PTV can be considered a 3D envelope in which the tumor and any microscopic extensions reside and move within this envelope. Once the PTV is defined, appropriate beam sizes to account for penumbra and beam arrangements must be selected to ensure the desired dose coverage of the PTV.
Report 50 refined the definition of organs at risk as normal tissues whose radiation sensitivity may significantly influence treatment planning and/or prescribed dose. The report did state that any possible movement of the organ at risk during treatment, as well as uncertainties in the setup during the whole treatment course, must be considered, but did not provide a method to do so. The hot spot definition was modified to be a volume outside the PTV that received a dose larger than 100 % of the specified PTV dose. This was considered clinically meaningful only if the minimum diameter exceeded 15 mm.
Report 50 was well suited to conformal therapy; however, its use raised new questions and prompted numerous publications [20, 21]. Also, as previously pointed out, irradiation techniques continued to evolve (e.g., IMRT), and advances in imaging procedures (e.g., PET, MRI) provided even more information on functionality; the location, shape, and limits of tumor/target volumes; and organs at risk. In response to these developments, the ICRU in 1999 published Report 62 [22], which expanded on some of the definitions and concepts of Report 50 and took into account the consequences of the technical and clinical progress, referred to previously. ICRU Report 62 refined the definition of PTV by introducing the concept of an internal margin to take into account variations in size, shape, and position of the CTV in reference to the patient’s coordinate system using anatomic reference points and also the concept of a setup margin to take into account all uncertainties in patient-beam positioning in reference to the treatment machine coordinate system. Setup margin uncertainties are related largely to technical factors that can be dealt with by more accurate setup and immobilization of the patient and improved mechanical stability of the machine. ICRU Report 62 refined the definition of the two dose volumes defined ICRU Report 50 as follows: The treated volume is the tissue volume that (according to the approved treatment plan) is planned to receive at least a dose selected and specified by radiation oncology team as being appropriate to achieve the purpose of the treatment, e.g., tumor eradication or palliation, within the bounds of acceptable complications. The irradiated volume is the tissue volume that receives a dose that is considered significant in relation to normal tissue tolerance.
Report 62 refined the definition of organs at risk as normal tissues (e.g., spinal cord) whose radiation sensitivity may significantly influence treatment planning and/or prescribed dose. Report 62 also addressed what was perhaps the most criticized limitation of Report 50, which was that it did not provide a method to account for organ at risk movements and changes in shape and/or size, as well as setup uncertainties. To account for such spatial uncertainties, Report 62 introduced the concept of the planning organ at risk volume (PRV), in which a margin is added around the organ at risk to compensate for that organ’s geometric uncertainties. The PRV margin around the organ at risk is analogous to the PTV margin around the CTV. The introduction of the PRV concept is timely as its use is even more important for those conformal therapy cases involving IMRT because of the increased sensitivity of this type treatment to geometric uncertainties.
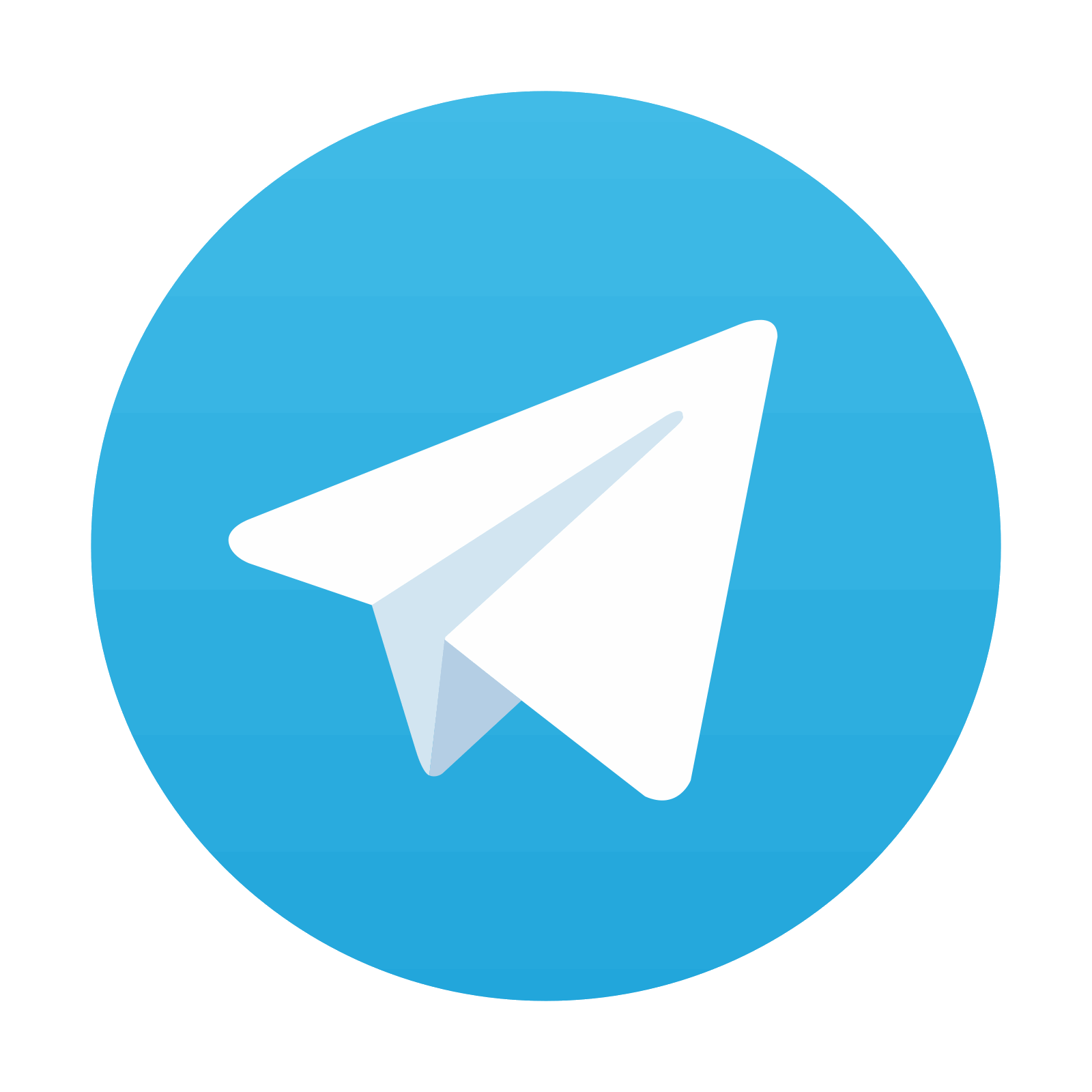
Stay updated, free articles. Join our Telegram channel
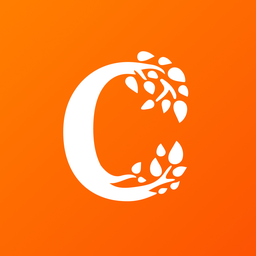
Full access? Get Clinical Tree
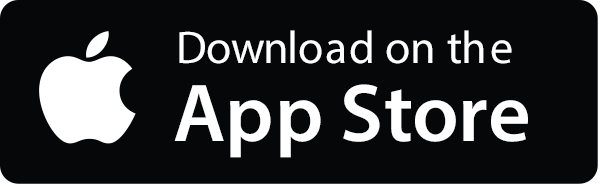
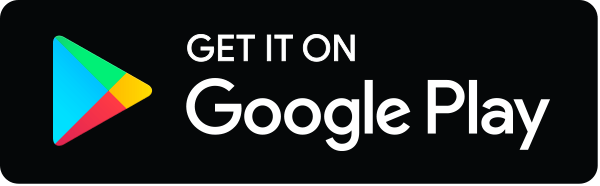