Repairing the Damaged Brain
Damage to Axons Affects Neurons and Neighboring Cells
Central Axons Regenerate Poorly After Injury
Therapeutic Interventions May Promote Regeneration of Injured Central Neurons
Environmental Factors Support the Regeneration of Injured Axons
Formation of New Connections by Intact Axons Can Lead to Functional Recovery
Neurons in the Injured Brain Die but New Ones Can Be Born
Therapeutic Interventions May Retain or Replace Injured Central Neurons
Transplantation of Neurons or Their Progenitors Can Replace Lost Neurons
Stimulation of Neurogenesis in Regions of Injury May Contribute to Restoring Function
Transplantation of Nonneuronal Cells or Their Progenitors Can Improve Neuronal Function
Restoration of Function Is the Aim of Regenerative Therapies
FOR MUCH OF ITS HISTORY NEUROLOGY has been a discipline of outstanding diagnostic rigor but little therapeutic efficacy. Simply put, neurologists have been renowned for their ability to localize lesions with great precision but until recently have had little to offer in terms of treatment. During the past decade this situation has begun to change.
Advances in our understanding of the structure, function, and chemistry of the brain’s neurons, glial cells, and synapses have led to new ideas for treatment. Many of these are now in clinical trials and some are already available to patients. Developmental neuroscience is emerging as a major contributor to this sea change for three main reasons. First, efforts to preserve or replace neurons lost to damage or disease rely on recent advances in our understanding of the mechanisms that control the generation and death of nerve cells (see Chapters 52 and 53). Second, efforts to improve the regeneration of neural pathways following injury draw heavily on what we have learned about the growth of axons and the formation of synapses (see Chapters 54 and 55). Third, there is increasing evidence that some devastating brain disorders, such as autism and schizophrenia, are the result of disturbances in the formation of neural circuits in embryonic or early postnatal life. Accordingly, studies of normal development may provide an essential foundation for discovering precisely what has gone wrong in disease.
In this chapter we focus on the first two of these issues: How neuroscientists hope to augment the limited ability of neurons to recover normal function. We shall begin by describing how axons degenerate following the separation of the axon and its terminals from the cell body. The regeneration of severed axons is robust in the peripheral nervous system of mammals and in the central nervous system of lower vertebrates, but very poor in the central nervous system of mammals. Many investigators have sought the reasons for these differences in the hope that understanding them will lead to methods for augmenting recovery of the human brain and spinal cord following injury. Indeed, we shall see that several differences in regenerative capacity of mammalian neurons have been discovered, each of which has opened promising new approaches to therapy.
Next we shall consider an even more dire consequence of neural injury: the death of neurons. The inability of the adult brain to form new neurons has been a central dogma of neuroscience since the pioneering neuroanatomist Santiago Ramón y Cajal asserted that in the injured central nervous system, “Everything may die, nothing may be regenerated.” This pessimistic view dominated neurology for most of the last century despite the fact that Ramón y Cajal added, “It is for the science of the future to change, if possible, this harsh decree.” Remarkably, in the past few decades evidence has accumulated that neurogenesis does occur in certain regions of the adult mammalian brain. This discovery has helped accelerate the pace of research on ways to stimulate neurogenesis and to replace neurons following injury. Although this work is preliminary, and in some respects controversial, it now seems possible that in the future neuroscientists will be able to reverse Cajal’s “harsh decree.”
Damage to Axons Affects Neurons and Neighboring Cells
Because neurons have very long axons and cell bodies of modest size, most injuries to the central or peripheral nervous system involve damage to axons. Transection of the axon, either by cutting or by crushing, is called axotomy, and its consequences are numerous.
Axon Degeneration Is an Active Process
Axotomy divides the axon in two: a proximal segment that remains attached to the cell body and a distal segment that has lost this crucial attachment. Axotomy dooms the distal segment of the axon. Synaptic transmission soon fails at severed nerve terminals. After a delay, physical degeneration of the axon occurs and once it begins its progression is relatively rapid and inevitably proceeds to completion (Figure 57-1). Within the distal segment the neuronal membrane breaks down, the cytoskeleton is disassembled, and cytoskeletal components are degraded. This degenerative response is the first step in an elaborate constellation of changes that were initially described in 1850 by Augustus Waller, changes that are now called Wallerian degeneration.
Figure 57-1 Axotomy affects the injured neuron and its synaptic partners.
A. A normal neuron with an intact functional axon wrapped by myelinating cells contacts a postsynaptic neuron. The neuron’s cell body is itself a postsynaptic target.
B. After axotomy the nerve terminals of the injured neuron begin to degenerate (1). The distal axonal stump separates from the parental cell body, becomes irregular, and undergoes Wallerian degeneration (2). Myelin begins to fragment (3) and the lesion site is invaded by phagocytic cells (4). The cell body of the damaged neuron undergoes chromatolysis: The cell body swells and the nucleus moves to an eccentric position (5). Synaptic terminals that contact the damaged neuron withdraw and the synaptic site is invaded by glial cell processes (6). The injured neuron’s inputs (7) and targets (8) can atrophy and degenerate.
The degeneration of transected axons was long thought to be a passive process, the consequence of separation from the neuronal cell body, within which most of the cell’s proteins are synthesized. Lacking a source of new protein, the distal stump would, in essence, wither away. But the discovery and analysis in mice of a spontaneously occurring mutant called Wlds (Wallerian degeneration slow) challenged this view (Figure 57-2). In the Wlds mutant the distal stumps of peripheral nerves persist for several weeks after transection, about 10-fold longer than in normal mice.
Figure 57-2 Axonal degeneration is delayed in Wlds mutant mice. After sectioning of a peripheral nerve in wild type animals, axons in the distal stump degenerate rapidly, as shown by disrupted axonal fragments (yellow) and the lack of myelinated axonal profiles at the electron micrographic level. In Wlds mutant mice the distal portion of severed axons persists for a long time. (Top two confocal micrographs reproduced, with permission, from Beirowski et al. 2004. Bottom two electron micrographs reproduced, with permission, from Michael Coleman.)
The Wlds mutation results in the fusion of two normal proteins into a novel protein. One of the contributing proteins is normally involved in biosynthesis of a metabolic cofactor, nicotinamide adenine dinucleotide (NADH), and the other is normally involved in ubiquitination, the process that covalently modifies proteins for degradation.
It remains unclear how the presence of the Wlds fusion protein slows degeneration so dramatically, but its discovery has been important in several ways. First, the very fact that degeneration can be slowed proves that it is not a passive consequence of separation from the cell body, but is rather an actively regulated response. Second, the nature of the Wlds protein has already given us clues about the nature of the active process that drives Wallerian degeneration.
Third, and perhaps most importantly, insight into how the Wlds fusion protein acts may be useful in devising treatments for neurological disorders in which axonal degeneration is prominent. A fatal disease of motor neurons, amyotrophic lateral sclerosis, falls into this category. Other possibilities include some forms of spinal muscular atrophy, Parkinson disease, and even Alzheimer disease. Axon degeneration that occurs in these diseases, as well as after metabolic, toxic, or inflammatory insults, resembles the degeneration that follows acute trauma and may be regulated in similar ways. Expression of the Wlds fusion protein significantly delays axonal loss and can even extend the life span of certain mouse models of motor neuron disease. Thus, while methods for saving transected distal axons are unlikely to be useful clinically for treating patients who have suffered traumatic injury, the same techniques could be useful in treating neurodegenerative diseases.
We return now to the injured axon itself. Even though the proximal portion of the axon remains attached to the cell body, it too suffers. And in some cases the neuron itself dies by apoptosis, probably because axotomy isolates the neuronal cell body from its supply of target-derived trophic factors. Even when this does not occur, the cell body often undergoes a series of cellular and biochemical changes called the chromatolytic reaction: The cell body swells, the nucleus moves to an eccentric position, and the rough endoplasmic reticulum becomes fragmented (Figure 57-1B). Chromatolysis is accompanied by other metabolic changes, including an increase in protein and RNA synthesis as well as a change in the pattern of genes that the neuron expresses. These changes are reversible if regeneration is successful.
Axotomy Leads to Reactive Responses in Nearby Cells
Axotomy sets in motion a cascade of responses in numerous types of neighboring cells. Among the most important responses are those of the glial cells that ensheath the distal nerve segment. The myelin sheath becomes fragmented and eventually removed.
This process is rapid in the peripheral nervous system, where the myelin-producing Schwann cells break the myelin into small fragments and engulf it. Schwann cells, which then divide, secrete factors that recruit macrophages from the blood stream. The macrophages in turn assist in the disposal of debris. Schwann cells also produce growth factors that promote axon regeneration, a point to which we will return later.
In contrast, in the central nervous system the myelin-forming oligodendrocytes have little or no ability to dispose of myelin, and the blood-brain barrier prevents the entry of macrophages, so removal of debris depends on a limited quantity of resident macrophages called microglia. These differences in cellular properties explain the observation that Wallerian degeneration proceeds to completion much more slowly in the central nervous system.
Axotomy also affects postsynaptic neurons. When axotomy disrupts the major inputs to a cell—as happens in denervated muscle, or to neurons in the lateral geniculate when the optic nerve is cut—the consequences are severe. Usually the target atrophies and sometimes dies. When targets are only partially denervated, their responses are more limited. In addition, axotomy affects presynaptic neurons. In many instances synaptic terminals withdraw from the cell body or dendrites of chromatolytic neurons and are replaced by the processes of glial cells—Schwann cells in the periphery and microglia or astrocytes in the central nervous system. This process, called synaptic stripping, depresses synaptic activity and can impair functional recovery.
Although the mechanism of synaptic stripping remains unclear, two possibilities have been suggested. One is that postsynaptic injury causes axon terminals to lose their adhesiveness to synaptic sites so that they are subsequently wrapped by glia. The other is that glia initiate the process of synaptic stripping in response to factors released from the injured neuron or to changes in its cell surface. Whatever the trigger, the activation of microglia and astrocytes by axotomy clearly contributes to the stripping process. In addition, biochemically altered astrocytes, called reactive astrocytes, contribute to formation of a glial scar near sites of injury.
As a result of these transsynaptic effects, neuronal degeneration can propagate through a circuit in both anterograde and retrograde directions. For example, a denervated neuron that becomes severely atrophic can fail to activate its target, which in turn becomes atrophic. Likewise, when synaptic stripping prevents an afferent neuron from obtaining sufficient sustenance from its target cell, the afferent neuron’s inputs are placed at risk. Such chain reactions help to explain how injury to one site in the central nervous system eventually affects regions far from the source of the injury.
Central Axons Regenerate Poorly After Injury
Central and peripheral nerves differ substantially in their ability to regenerate after injury. Peripheral nerves can often be repaired following injury. Although the distal segments of axons degenerate, connective tissue elements surrounding the distal stump generally survive. Axonal sprouts grow from the proximal stump, enter the distal stump, and grow along the nerve toward its targets (Figure 57-3). The mechanisms that drive this process are related to those that guide embryonic axons. Chemotropic factors secreted by Schwann cells attract axons to the distal stump, adhesive molecules within the distal stump promote axon growth along cell membranes and extracellular matrices, and inhibitory molecules in the perineural sheath prevent regenerating axons from going astray.
Figure 57-3 Axons in the periphery regenerate better than those in the central nervous system. After sectioning of a peripheral nerve, the perineural sheath reforms rapidly and Schwann cells in the distal stump promote axonal growth by producing trophic and attractant factors and expressing high levels of adhesive proteins. After sectioning of axonal tracts in the central nervous system, the distal segment disintegrates and myelin fragments. In addition, reactive astrocytes and macrophages are attracted to the lesion site. This complex cellular milieu, termed a glial scar, inhibits axonal regeneration.
Once regenerated peripheral axons reach their targets they are able to form new functional nerve endings. Motor axons form new neuromuscular junctions; autonomic axons successfully reinnervate glands, blood vessels, and viscera; and sensory axons reinnervate muscle spindles. Finally, those axons that lost their myelin sheaths are remyelinated, and chromatolytic cell bodies regain their original appearance. Thus in all three divisions of the peripheral nervous system—motor, sensory, and autonomic—the effects of axotomy are reversible. This is not to imply that peripheral regeneration is perfect. In the motor system recovery of strength may be substantial, but recovery of fine movements is usually impaired. Some motor axons form synapses on inappropriate muscle fibers, some peripheral axons never find their targets, and some neurons die. Nevertheless, the regenerative capacities in the peripheral nervous system are impressive.
In contrast, in the central nervous system regeneration after injury is poor (Figure 57-3). The proximal stumps of damaged axons can form short sprouts, but these soon stall and form swollen endings called “retraction bulbs” that fail to progress. Long-distance regeneration is rare. The longstanding failure of central regeneration has led to the pessimistic view that injuries to the brain and spinal cord are largely irreversible, and that therapy must be restricted to rehabilitative measures.
For some time neurobiologists have been seeking the reasons why regenerative capacity in the central and peripheral nervous systems differs so dramatically. The goal of this work is to identify the crucial barriers to regeneration so that they can be overcome. These studies have begun to bear fruit, and there is now cautious optimism that the injured human brain and spinal cord have a regenerative capacity that can eventually be exploited.
Before discussing these new developments it is helpful to consider the problem of neural regeneration in a broader biological context. Is it the ability of peripheral axons to regenerate that is unusual, or the inability of central axons to do so? It is in fact the latter. Obviously, central axons grow well during development. More surprisingly, axons in immature mammals can also regenerate following transection in the brain or spinal cord. Moreover, regeneration is robust in the central nervous systems of lower vertebrates such as fish and frogs, as exemplified by the studies of Roger Sperry on restoration of vision following damage to the optic nerve (see Chapter 54).
So why have mature mammals lost this seemingly important capacity for repair? The answer may lie in what the mammalian brain can do peerlessly, which is to remodel its basic wiring diagram in accordance with experience during critical periods in early postnatal life, so that each individual’s brain is optimized to deal with the changes and challenges of internal and external worlds (see Chapter 56). But once remodeling has occurred, it must be stabilized. It is obviously useful to reassign cortical space to one eye if the other is blinded in childhood, but we would not want our cortical connections similarly rearranged in response to a brief period of unusual illumination or darkness. Maintaining constancy in the face of small perturbations in connectivity may therefore have the unavoidable consequence of limiting the ability of central connections to regenerate in response to injury. In this view our limited regenerative capacity is the Faustian biological bargain we have made for the possession of many precisely wired circuits that underlie our superior intellectual powers.
Therapeutic Interventions May Promote Regeneration of Injured Central Neurons
In seeking reasons for the poor regeneration of central axons, one critical question is whether the poor recovery reflects an inability of neurons themselves to grow or an inability of the environment to support axonal growth. This issue was addressed by Albert Aguayo and his colleagues in the early 1980s. They inserted segments of a central nerve trunk into a peripheral nerve graft, and segments of a peripheral nerve into the brain or spinal cord, to find out how the translocated axons would respond.
Aguayo found that the axons in the translocated segments promptly degenerated, leaving “distal stumps” containing glia, support cells, and extracellular matrix. The results were striking. Spinal axons that regenerated poorly following spinal cord injury grew several centimeters when inserted into a peripheral nerve (Figure 57-4). Conversely, peripheral axons regenerated well through their own distal nerve trunk, but fared poorly when paired with a severed optic nerve (Figure 57-5).
Figure 57-4 A transplanted peripheral nerve provides a favorable environment for the regeneration of central axons. Left: After sectioning of the spinal cord, ascending and descending axons fail to cross the lesion site. Right: Insertion of a bridging peripheral nerve graft that bypasses the lesion site promotes regeneration of both ascending and descending axons. (Adapted, with permission, from Aguayo 1981.)
Figure 57-5 Peripheral and central nerves differ in their ability to support axonal regeneration.
A. In the peripheral nervous system severed axons regrow past the site of injury. Insertion of a segment of optic nerve into a peripheral nerve suppresses the ability of the peripheral nerve to regenerate.
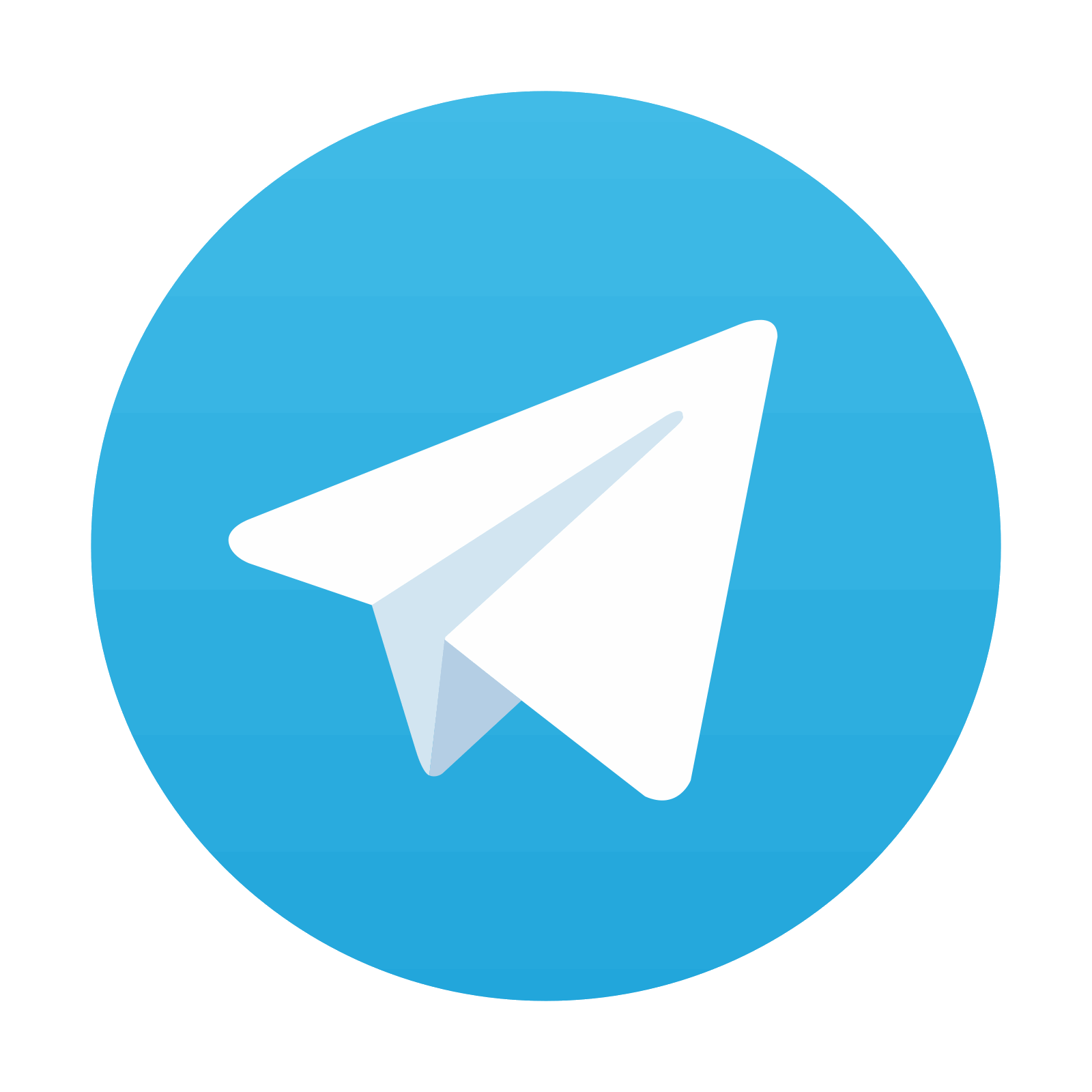
Stay updated, free articles. Join our Telegram channel
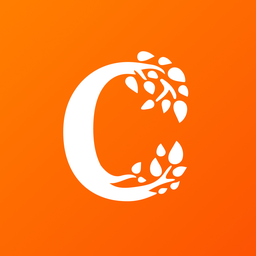
Full access? Get Clinical Tree
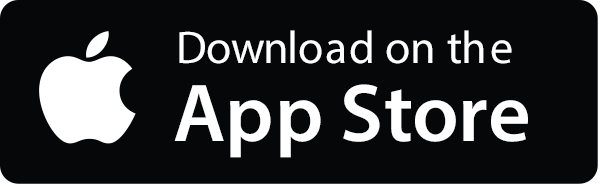
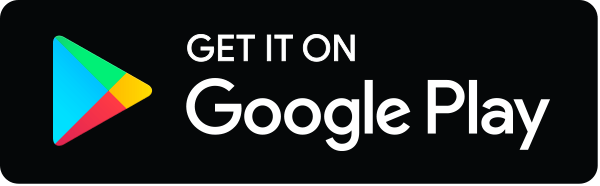