Review of Basic Circuit Theory
Current in Circuits with Capacitance
FAMILIARITY WITH THE BASIC PRINCIPLES of electrical circuit theory is important for understanding the equivalent circuit model of the neuron developed in Chapters 6, 7, and 9. The appendix is divided into three parts:
1. The definition of basic electrical parameters.
2. A set of rules for elementary circuit analysis.
3. A description of current in circuits with capacitance.
Basic Electrical Parameters
Potential Difference (V or E)
Electrical charges exert an electrostatic force on other charges: like charges repel, opposite charges attract. The force decreases as the distance between two charges increases. Work is done when two charges that initially are separated are brought together. Negative work is done if their polarities are opposite and positive work if they are the same. The greater the values of the charges and the greater their initial separation, the greater the work done. (Work where f is electrostatic force and r1 is the initial distance between the two charges.)
Potential difference is a measure of this work: The potential difference between two points is the work that must be done to move a unit of positive charge (one coulomb) from one point to the other (ie, it is the potential energy of the charge). One volt (V) is the energy required to move one coulomb a distance of one meter against a force of one newton.
Current (I)
A potential difference exists within a system whenever positive and negative charges are separated. Charge separation may be generated by a chemical reaction (as in a battery) or by diffusion of two electrolyte solutions with different ion concentrations across a selectively permeable barrier, such as a cell membrane. If a charge separation exists within a conducting medium, charges move between the areas of potential difference: Positive charges are attracted to the region with a more negative potential, and negative charges to the region of positive potential.
Current is defined as the net movement of charge per unit time. According to convention, the direction of current is defined as the direction of flow of positive charge. In metallic conductors current is carried by negatively charged electrons, which move in the opposite direction of conventionally defined current. In nerve and muscle cells current is carried by both positive and negative ions in solution. One ampere (A) of current represents the movement of one coulomb (of charge) per second.
Conductance (g)
Any object through which electrical charges can flow is called a conductor. The unit of electrical conductance is the siemens (S). According to Ohm’s law the current that flows through a conductor is directly proportional to the potential difference across it:1
As charge carriers move through a conductor, some of their electrical potential energy is converted into thermal energy caused by their frictional interactions with the conducting medium.
Each type of material has an intrinsic property called conductivity (σ), which is determined by its molecular structure. Metallic conductors conduct electricity extremely well and thus have high conductivities. Aqueous solutions with high-ionized salt concentrations have somewhat lower conductivity, and lipids have very low conductivity—they are poor conductors of electricity and are therefore good insulators. The conductance of an object is proportional to σ times its cross-sectional area divided by its length:
Length is defined as the direction along which one measures conductance. For example, the conductance measured along the cytoplasmic core of an axon is reduced if its length is increased or its diameter decreased (Figure A–1).
Figure A-1 The conductance of an object is determined by geometric factors, together with conductivity. Conductance is inversely proportional to the length of the conductor (A) and directly proportional to the cross-sectional area of the conductor (B).
Electrical resistance (R) is the reciprocal of conductance and a measure of the resistance provided by an object to current. Resistance is measured in ohms (Ω):
Capacitance (C)
A capacitor consists of two conducting plates separated by an insulating layer. Its fundamental property is its ability to separate charges of opposite sign: Positive charges are stored on one plate, negative charges on the other. In the example in Figure A–2 a net excess of positive charges on plate x and an equal excess of negative charges on plate y results in a potential difference between the two plates.
Figure A-2 The capacitance of a parallel-plate capacitor is determined by the area of the two plates and the distance between them.
A. A test charge moved between two charged plates must overcome a force. The work done against this force is the potential difference between the two plates.
B. Increasing the density of charge carriers increases the potential difference.
C. Increasing the area of the plates increases capacitance by increasing the number of charges required to produce a given potential difference.
D. Increasing the distance between the two plates decreases capacitance, decreasing the number of charges required to produce a given potential difference.
This potential difference can be measured by determining how much work is required to move a positive test charge from y to x. Initially, the test charge is attracted by the negative charges on y and repelled by the more distant positive charges on x. The result of these electrostatic interactions is a force f that opposes the movement of the charge from y to x. However, as the test charge is moved toward x, the attraction by the negative charges on y diminishes and the repulsion by the positive charges on x increases, with the result that the net electrostatic force exerted on the test charge is constant everywhere between x and y. Work (W) is force times the distance (D) over which the force is exerted:
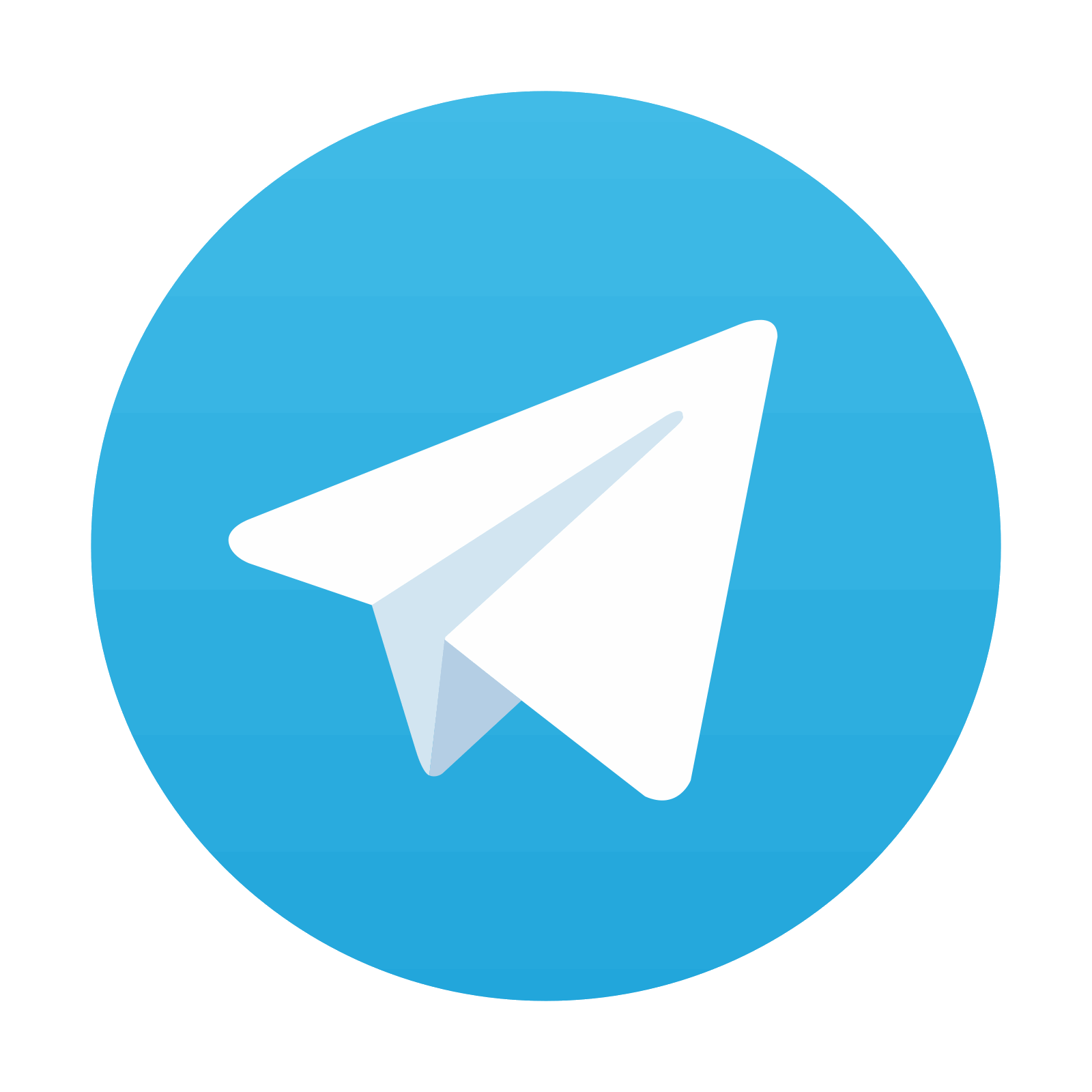
Stay updated, free articles. Join our Telegram channel
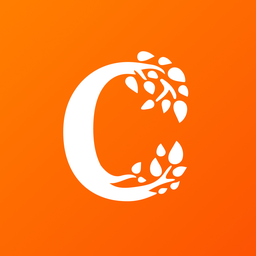
Full access? Get Clinical Tree
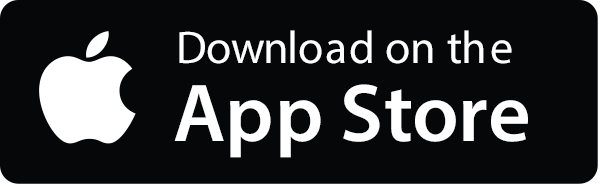
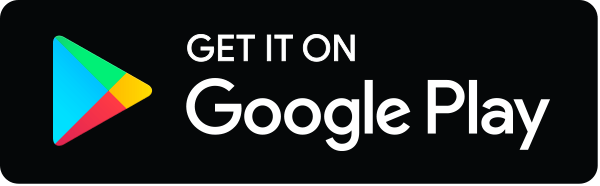