Seizures and Epilepsy
Classification of Seizures and the Epilepsies Is Important for Pathogenesis and Treatment
The Electroencephalogram Represents the Collective Behavior of Cortical Neurons
Focal Seizures Originate Within a Small Group of Neurons Known as a Seizure Focus
The Breakdown of Surround Inhibition Leads to Synchronization
The Spread of Focal Seizures Involves Normal Cortical Circuitry
Primary Generalized Seizures Are Driven by Thalamocortical Circuits
Locating the Seizure Focus Is Critical to the Surgical Treatment of Epilepsy
Prolonged Seizures Can Cause Brain Damage
The Factors Leading to Development of Epilepsy Are an Unfolding Mystery
Among the Genetic Causes of Epilepsy Are Ion Channel Mutations
Epilepsies Involving Focal Seizures May Be a Maladaptive Response to Injury
UNTIL QUITE RECENTLY THE FUNCTION and organization of the human cerebral cortex—the structure of the brain concerned with perceptual, motor, and cognitive functions—has eluded both clinicians and neuroscientists. In the past the analysis of brain function relied in large part on observations of the behavioral consequences of brain damage caused by strokes or trauma. These natural experiments provided much of the early evidence that distinct brain regions serve specific functions (see Chapter 1).
Observation of patients with seizures and epilepsy has been equally important in the study of brain function because the behavioral consequences of these disorders vary with the brain regions from which they originate. Seizures are temporary disruptions of brain function resulting from abnormal, excessive neuronal activity; epilepsy is a chronic condition of repeated seizures. For centuries understanding the neurological origins of seizures was confounded by the dramatic, sometimes bizarre behaviors associated with seizures. Epilepsy was widely associated with possession by evil spirits, while seizures were thought to reflect oracular, prescient, or special creative powers.
The Greeks in the time of Hippocrates (circa 400 bc) were aware that head injuries to one side of the brain could cause seizure activity on the opposite side of the body. In those earlier times the diagnosis of epilepsy was probably much broader than the contemporary definition. Other causes of episodic unconsciousness, such as syncope as well as mass hysteria and psychogenic seizures, were almost certainly classified with epilepsy. Moreover, historical writings typically describe generalized convulsive seizures involving both cerebral hemispheres. Thus it is likely that focal seizures involving a limited area of the brain were misdiagnosed or never diagnosed at all. Even today it can be difficult for physicians to distinguish between episodic loss of consciousness and the various types of seizures. Nevertheless, as our ability to treat and even cure epilepsy continues to improve, these diagnostic distinctions take on increasing significance.
The modern neurobiological analysis of epilepsy began with John Hughlings Jackson’s work in London in the 1860s. Jackson realized that seizures need not involve loss of consciousness but could be associated with localized symptoms such as the jerking of an arm. His observation was the first formal recognition of what we now call focal (or partial) seizures. Jackson also observed patients whose seizures began with focal neurological symptoms and progressed to convulsions with loss of consciousness (the so-called Jacksonian march).
Another early development that presaged modern therapy was the first surgical treatment for epilepsy in 1886 by Victor Horsley. Horsley resected cerebral cortex adjacent to a depressed skull fracture and cured a patient with focal motor seizures. The modern surgical treatment for epilepsy dates to the work of Wilder Penfield and Herbert Jasper in Montreal in the early 1950s. Medical innovations include the first use of phenobarbital as an anticonvulsant in 1912 by Alfred Hauptmann, the development of electroencephalography by Hans Berger in 1929, and the discovery of the anticonvulsant properties of phenytoin (Dilantin) by Houston Merritt and Tracey Putnam in 1937.
As in any chronic disease, the physiological features of seizures are not the only consideration in the care and management of patients with epilepsy. Psychosocial factors are also extremely important. The diagnosis of epilepsy has consequences that can affect all aspects of everyday life, including educational opportunities, driving, and employment. Although many limitations imposed on epileptics are appropriate—most would agree that patients with epilepsy should not be commercial pilots—a diagnosis of epilepsy can have inappropriate, negative effects on educational opportunities and employment. To improve this situation, physicians have a duty to educate themselves and the public.
Classification of Seizures and the Epilepsies Is Important for Pathogenesis and Treatment
Not all seizures are the same. Thus the pathology of seizures must take into account their clinical features. Seizures, and the chronic condition of repetitive seizures (epilepsy), are common. Based on epidemiological studies in the United States, somewhere around 3% of all individuals living to the age of 80 years are diagnosed with epilepsy. The highest incidence occurs in young children and the elderly.
In many respects seizures represent a prototypic neurological disease in that the symptoms include both positive and negative sensory or motor manifestations. Examples of positive signs that can occur during a seizure include the perception of flashing lights or the jerking of an arm. Negative signs such as impairment of consciousness and self-awareness or even transient blindness or paralysis reflect impairment of normal brain function. These examples underscore a general feature of seizures: The signs and symptoms depend on the location and extent of brain regions that are affected. Finally, the manifestations of seizures result in part from the activity in normal tissue with normal cellular and network properties. The latter is particularly important in the spread of a focal or partial seizure beyond its original boundaries. Seizures quite literally hijack the normal functions of the brain.
Seizures Are Temporary Disruptions of Brain Function
Seizures can be classified conceptually into two categories: focal (or partial) and generalized (Table 50-1). Although the details of seizure classification are under continuous discussion, this simple dichotomy has proven extremely useful to clinicians because anticonvulsant medications often target preferentially to one or the other type of seizure. The terms “focal” and “partial” are used interchangeably in this chapter.
Table 50-1 International League Against Epilepsy (ILAE) Classification of Seizures
Focal seizures originate in a small group of neurons (the seizure focus) and thus the symptoms depend on the location of the focus within the brain. Focal seizures were formerly classified as simple partial when there is no alteration of consciousness, or complex partial when there is an alteration of consciousness. A typical focal seizure might begin with jerking in the right hand and progress to clonic movements (ie, jerks) of the entire right arm. This seizure could also be called a focal motor seizure. If a focal seizure progresses further the patient may lose consciousness, fall to the ground, rigidly extend all extremities (tonic phase), then have jerking in all extremities (clonic phase). In this case, the focal seizure has secondarily generalized.
The onset of a focal seizure is often preceded by symptoms called auras. Common auras include abnormal sensations such as a sense of fear, a rising feeling in the abdomen, or even a specific odor. The aura is caused by electrical activity originating from the seizure focus and thus represents the earliest manifestations of a focal seizure. The time after a seizure but before the patient returns to his or her normal level of neurological function is called the postictal period.
Generalized seizures constitute the second main category. They begin without an aura or focal seizure and involve both hemispheres from the onset. Thus they are sometimes called primary generalized seizures to avoid confusion with seizures that begin from a focus and then generalize secondarily. Primary generalized seizures can be further divided into convulsive or nonconvulsive types depending on whether the seizure is associated with tonic or clonic movements.
The prototypic nonconvulsive generalized seizure is the typical absence seizure in children (formerly called petit mal). These seizures begin abruptly, usually last less than 10 seconds, are associated with cessation of all motor activity, and result in loss of consciousness but not loss of posture. The patients appear as if in a trance, but the episodes are so brief that their occurrence can be missed by a casual observer. Unlike a focal seizure there is no aura before the seizure or confusion after the seizure (the postictal period). Patients may exhibit mild motor manifestations such as eye blinking but do not fall or have tonic-clonic movements. Typical absence seizures have very distinctive electrical characteristics on the electroencephalogram (EEG).
Other types of generalized seizures can involve only abnormal movements (myoclonic, clonic, or tonic) or a sudden loss of motor tone (atonic). The most common convulsive generalized seizure is the tonic-clonic (formerly called grand mal seizure). Such seizures begin abruptly, often with a grunt or cry, as tonic contraction of the diaphragm and thorax forces expiration. During the tonic phase the patient may fall to the ground in a rigid posture with clenched jaw, lose bladder or bowel control, and become blue (cyanotic). The tonic phase typically lasts 30 seconds before evolving into clonic jerking of the extremities lasting 1 to 2 minutes. This active phase is followed by a postictal phase during which the patient is sleepy and may complain of a headache and muscle soreness.
A primary generalized tonic-clonic seizure can be difficult to distinguish on purely clinical grounds from a secondarily generalized tonic-clonic seizure with a brief aura. This distinction is not simply academic; it can be vital to choosing proper treatment as well as pinpointing the underlying cause.
Epilepsy Is the Chronic Condition of Recurrent Seizures
Recurrent seizures constitute the minimal criterion for the diagnosis of epilepsy. The oft-quoted clinical rule emphasizes this point: “A single seizure does not epilepsy make.” Various factors that contribute to a clinical pattern of recurrent seizures—the underlying etiology of the seizures, the age of onset, or family history—are ignored in the seizure classification scheme in Table 50-1. The classification of the epilepsies initially evolved primarily based upon clinical observation rather than a precise cellular, molecular, or genetic understanding of the disorder. The factors influencing seizure type and severity were sometimes recognized as patterns of symptoms, referred to as the epilepsy syndromes.
In the 1989, according to the International League Against Epilepsy (ILAE) classification of the epilepsies, the primary variables were whether or not a focal brain abnormality could be identified (localization-related versus generalized epilepsies), and whether or not a cause could be identified (symptomatic versus idiopathic). Most adult-onset epilepsies could be classified as symptomatic localization-related epilepsy, a category that included such causes as trauma, stroke, tumors, and infections. However, many patients have adult-onset epilepsies without a clearly defined cause. Thus, despite the usefulness of this scheme, many types of epilepsy did not fit neatly into its categories. In 2010 the ILAE recommended focusing on the underlying causes of epilepsies based on three broad variables—genetic, structural/metabolic, and unknown—as well as a list of electroclinical syndromes. One expects and hopes that these classifications will be elaborated as knowledge of the underlying causes increases.
The Electroencephalogram Represents the Collective Behavior of Cortical Neurons
Because neurons are excitable cells, it should not be surprising that seizures result either directly or indirectly from a change in the excitability of single neurons or groups of neurons. This view dominated early experimental studies of seizures. Electrical recordings of brain activity can be made with intracellular or extracellular electrodes. Extracellular electrodes sense action potentials in nearby neurons and can detect the synchronized activity of ensembles of cells called field potentials.
At the slow time resolution of extracellular recording (hundreds of milliseconds to seconds) field potentials can appear as single transient changes called spikes. These spikes reflect action potentials in many neurons and should not be confused with spikes in single neurons, which are individual action potentials that last only 1 or 2 milliseconds. The EEG thus represents a set of field potentials as recorded by multiple electrodes on the surface of the scalp (Figure 50-1).
Figure 50-1 The normal electroencephalogram (EEG) in an awake human subject.
A. A standard set of placements (or montage) of electrodes on the surface of the scalp. The electrical response at each site reflects the activity between two of the electrodes.
B. At the beginning of the recording the EEG shows low-voltage activity (circa 20 μV) over the surface of the scalp. The vertical lines are placed at 1 second intervals. During the first 8 seconds the subject was resting quietly with eyes open, then the subject was asked to close his eyes. With the eyes closed, larger-amplitude activity (8-10 Hz) develops over the occipital region (sites 3, 4, 8, 12, 15, 16). This is the normal alpha rhythm characteristic of the relaxed, wakeful state. Slow large-amplitude artifacts occur at 3.5 seconds when the eyes blink and at 9 seconds when the eyes close.
Because the electrical activity originates in neurons in the underlying brain tissue, the waveform recorded by the surface electrode depends on the orientation and distance of the electrical source with respect to the electrode. The EEG signal is inevitably distorted by the filtering and attenuation caused by intervening layers of tissue and bone that act in the same way as resistors and capacitors in an electric circuit. Thus the amplitude of EEG signals (measured in microvolts) is much smaller than the voltage changes in a single neuron (measured in millivolts). High-frequency activity in single cells, such as action potentials, is filtered out by the EEG signal, which primarily reflects slower voltage changes across the cell membrane, such as synaptic potentials.
Although the EEG signal is a measure of the extracellular current caused by the summated electrical activity of many neurons, not all cells contribute equally to the EEG. The surface EEG reflects predominantly the activity of cortical neurons in close proximity to the EEG electrode. Thus deep structures such as the hippocampus, thalamus, or brain stem do not contribute directly to the surface EEG. The contributions of individual nerve cells to the EEG are discussed in Box 50-1.
The surface EEG shows patterns of activity—characterized by the frequency and amplitude of the electrical activity—that correlate with various stages of sleep and wakefulness (see Chapter 51), and with some pathophysiological processes such as seizures. The normal human EEG shows activity over the range of 1 to 30 Hz with amplitudes in the range of 20 to 100 μV. The observed frequencies have been divided into several groups: alpha (8-13 Hz), beta (13-30 Hz), delta (0.5-4 Hz), and theta (4-7 Hz).
Alpha waves of moderate amplitude are typical of relaxed wakefulness and are most prominent over parietal and occipital sites. During intense mental activity, beta waves of lower amplitude are more prominent in frontal areas and over other regions. Alerting relaxed subjects by asking them to open their eyes results in so-called desynchronization of the EEG with a reduction in alpha activity and an increase in beta activity (Figure 50-1B). Theta and delta waves are normal during drowsiness and early slow-wave sleep; if present during wakefulness, these waves are a sign of brain dysfunction.
As neuronal ensembles become synchronized, as when a subject relaxes or becomes drowsy, the summated currents become larger and can be seen as abrupt changes from the baseline activity. Such paroxysmal activity can be normal; for example, episodes of high-amplitude activity (1-2 s, 7-15 Hz) occur during sleep (sleep spindles). However, a sharp wave or EEG spike can also provide a clue to the location of a seizure focus in a patient with epilepsy (Figure 50-4).
Focal Seizures Originate Within a Small Group of Neurons Known as a Seizure Focus
Despite the variety of clinically defined seizures, important insights into the generation of seizure activity can largely be understood by comparing the electrographic patterns of focal and primary generalized seizures. The defining feature of focal (and secondarily generalized) seizures is that the abnormal electrical activity originates from a seizure focus. The seizure focus is nothing more than a small group of neurons, perhaps 1,000 or so, which have enhanced excitability and the ability to occasionally spread that activity to neighboring regions and thereby cause a seizure.
The enhanced excitability (epileptiform activity) may result from many different factors such as altered cellular properties or altered synaptic connections caused by a local scar, blood clot, or tumor. A discrete focus in the primary motor cortex may cause twitching of a finger or jerking of a limb (sometimes called simple partial seizure), whereas a seizure focus in the limbic system is frequently associated with unusual behaviors or an alteration of consciousness (sometimes called complex partial seizure).
The development of a focal seizure can be arbitrarily divided into four phases: (1) the interictal period between seizures followed by (2) synchronization of activity within the seizure focus (Figure 50-4), (3) seizure spread, and finally (4) secondary generalization. Phases 2 to 4 represent the ictal phase of the seizure. Different factors contribute to each phase.
Figure 50-4 The EEG can provide clues to the location of a seizure focus. Each trace represents the electrical activity between pairs of scalp electrodes as indicated in the electrode map. For example, electrode pairs 11-15 and 15-13 measure activity from the right temporal area. In the EEG record shown here, from a patient with epilepsy, sharp waves occur over the right temporal area (records enclosed in boxes). Such paroxysmal activity arises suddenly and disrupts the normal background EEG pattern. The focal abnormality may indicate that the seizure focus in this patient is in the right temporal lobe. Because the patient had no clinical seizures during the recording, these are interictal spikes (see Figure 50-7). (Adapted, with permission, from Lothman and Collins 1990.)
Figure 50-7 Interictal spikes as measured in the EEG result from the synchronized discharges of a group of hippocampal neurons. (Adapted, with permission, from Wong, Miles, and Traub 1984.)
A. Rhythmic firing is evident in an intracellular recording from a pyramidal cell in a hippocampal slice. An extracellular recording from the same slice shows the synchronized discharge of many neurons. This type of synchronized activity underlies interictal spikes in the EEG.
B. The hippocampal slice was perfused with bicuculline, which blocks the inhibition mediated by GABAA receptors in pyramidal cells and increases the occurrence of seizure-like activity. An intracellular recording from the slice shows several action potentials in one cell (top trace). On the next trial (lower trace) a hyperpolarizing current was injected to prevent the cell from firing, revealing the large paroxysmal depolarization shift that produces the sudden and long-lasting firing of neurons in a seizure focus.
Much of our knowledge about the electrical events during seizures comes from studies of animal models of focal seizures. A seizure is induced in an animal by focal electrical stimulation or by acute injection of a convulsant agent. This approach and the development of in vitro brain slice preparations (Box 50-2) have provided a good understanding of electrical events within the focus during a seizure as well as during the interictal period
Neurons in a Seizure Focus Have Characteristic Activity
How does electrical activity in a single neuron or group of neurons lead to a seizure? Each neuron within a seizure focus has a stereotypic and synchronized electrical response called the paroxysmal depolarizing shift, an intracellular depolarization that is sudden, large (20-40 mV), and long-lasting (50-200 ms), and triggers a train of action potentials at its peak (Figure 50-7B). The paroxysmal depolarizing shift is followed by an afterhyperpolarization.
The paroxysmal depolarizing shift and afterhyperpolarization are shaped by the intrinsic membrane properties of the neuron (eg, voltage-gated Na+, K+, and Ca2+ channels) and by synaptic inputs from excitatory and inhibitory neurons (primarily glutaminergic and GABAergic, respectively). The depolarizing phase results primarily from activation of AMPA- and NMDA-type glutamate receptor-channels (Figure 50-8A), as well as voltage-gated Na+, and Ca2+ channels. The NMDA-type receptor-channels are particularly suited to enhancing excitability because depolarization relieves Mg2+ blockage of the channel. Once unblocked, excitatory current through the channel increases, thus enhancing the depolarization and allowing extra Ca2+ to enter the neuron (see Chapter 10).
Figure 50-8 The conductances that underlie the paroxysmal depolarizing shift (PDS) of a neuron in a seizure focus.
A. The paroxysmal depolarizing shift is largely dependent on AMPA- and NMDA-type glutamate receptor-channels. The effectiveness of the NMDA-type is enhanced by the opening of voltage-gated Ca2+ channels (gCa). Following the depolarization the cell is hyperpolarized by activation of GABA receptors (both ionotropic GABAA and metabotropic GABAB) as well as by voltage-gated and calcium-activated K+ channels (gK). (AMPA α-amino-3-hydroxy-5-methylisoxazole-4-propionate; GABA, γ,-aminobutyric acid; NMDA, N-methyl-D-aspartate.) (Adapted, with permission, from Lothman 1993a.)
B. A simplified version of the inputs to a cortical pyramidal neuron. The orange terminals are excitatory, whereas the gray terminals are inhibitory. Recurrent axon branches activate inhibitory neurons, causing feedback inhibition of the pyramidal neuron. Extrinsic excitatory inputs can also activate feed-forward inhibition.
The normal response of a cortical pyramidal neuron to excitatory input consists of an excitatory postsynaptic potential (EPSP) followed by an inhibitory postsynaptic potential (IPSP) (because of the basic circuitry shown in Figure 50-8B). Thus the paroxysmal depolarizing shift can be viewed as a massive enhancement of the normal depolarizing and hyperpolarizing synaptic components. The afterhyperpolarization is generated by several types of K+ channels as well as a GABA receptor-mediated Cl− conductance (iono-tropic GABAA receptors) and K+ conductance (metabotropic GABAB receptors) (Figure 50-8A). The Ca2+ entry through voltage-dependent Ca2+ channels and NMDA-type receptor-channels triggers the opening of calcium-activated channels, particularly K+ channels. The afterhyperpolarization limits the duration of the paroxysmal depolarizing shift; its gradual disappearance is an important factor in the onset of a focal seizure, as discussed later.
Thus it is not surprising that many convulsants act by enhancing excitation or blocking inhibition. Conversely, anticonvulsants act by blocking excitation or enhancing inhibition. For example, the benzodiazepines diazepam (Valium) and lorazepam (Ativan) enhance GABAA-mediated inhibition and are used in the emergency treatment of prolonged repetitive seizures. The commonly used anticonvulsants phenytoin (Dilantin) and carbamazepine (Tegretol) cause reduction in the opening of the voltage-gated Na+ channels that underlie the action potential. The ability of these drugs to block the Na+ channels is enhanced by repetitive activity associated with seizures.
The Breakdown of Surround Inhibition Leads to Synchronization
As long as the abnormal electrical activity is restricted to a small group of neurons, there are no clinical manifestations. The synchronization of neurons in the focus is dependent not only on the intrinsic properties of each individual cell but also on the connections between neurons. During the interictal period the abnormal activity is confined to the seizure focus by inhibitory effects of the excited region on surrounding tissue. This inhibitory surround is particularly dependent on feed-forward and feedback inhibition by GABA-ergic inhibitory interneurons (Figure 50-9A).
Figure 50-9 The spatial and temporal organization of a seizure focus depends on the interplay between excitation and inhibition of neurons in the focus.
A. In this hypothetical seizure focus in the neocortex, the pyramidal cell a shows the typical electrical properties of neurons in a focus (see part B). Activity in cell a activates another pyramidal cell (b), and when many such cells fire synchronously a spike is recorded on the EEG. However, cell a also activates GABAergic inhibitory interneurons (gray). These interneurons can reduce the activity of cells a and b through feedback inhibition, thus limiting the seizure focus temporally, as well as prevent the firing of cells outside the focus, represented here by cell c. This latter phenomenon creates an inhibitory surround that contains the seizure focus spatially. When extrinsic or intrinsic factors alter this balance of excitation and inhibition, the inhibitory surround begins to break down and the seizure activity spreads. (Reproduced, with permission, from Lothman and Collins 1990.)
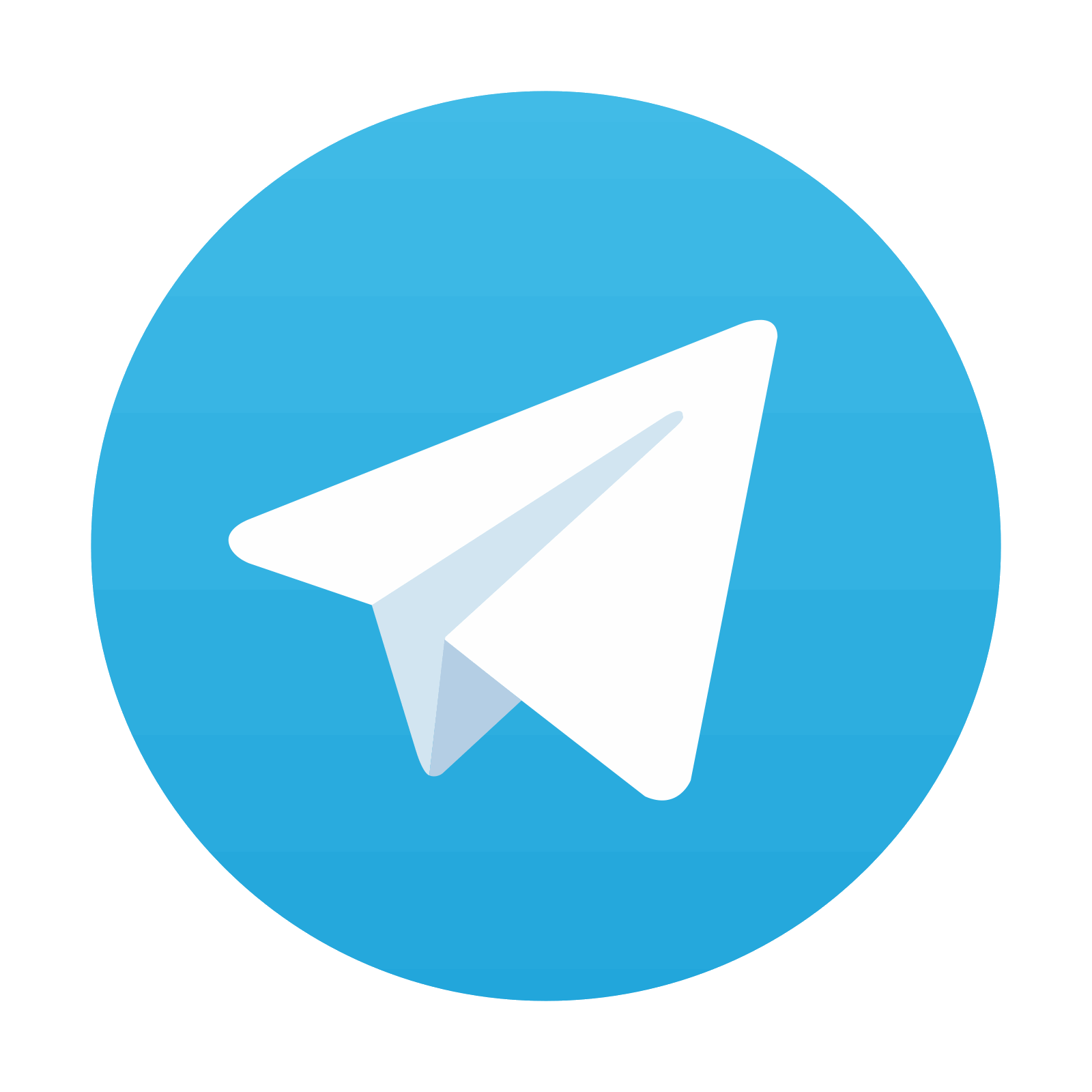
Stay updated, free articles. Join our Telegram channel
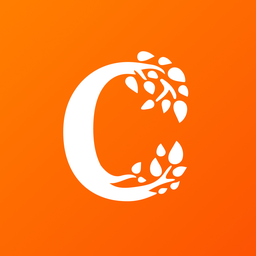
Full access? Get Clinical Tree
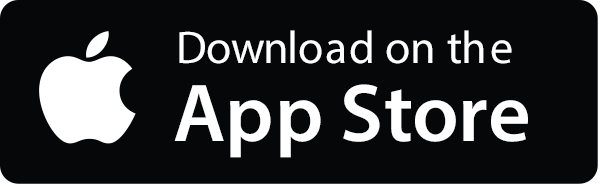
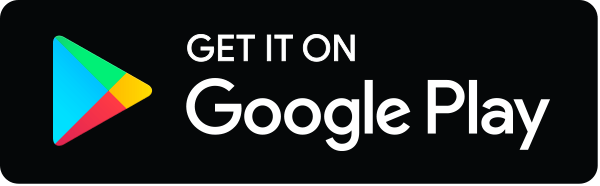