Signaling at the Nerve-Muscle Synapse: Directly Gated Transmission
The Neuromuscular Junction Is a Well-Studied Example of Directly Gated Synaptic Transmission
The Motor Neuron Excites the Muscle by Opening Ligand-Gated Ion Channels at the End-Plate
The End-Plate Potential Is Produced by Ionic Current Through Acetylcholine Receptor-Channels
The Ion Channel at the End-Plate Is Permeable to Both Sodium and Potassium
The Current Through Single Acetylcholine Receptor-Channels Can Be Measured Using the Patch Clamp
Individual Receptor-Channels Conduct All-or-None Unitary Currents
The Molecular Properties of the Acetylcholine Receptor-Channel Are Known
Postscript: The End-Plate Current Can Be Calculated from an Equivalent Circuit
COMMUNICATION BETWEEN NEURONS in the brain relies mainly on chemical synapses. Much of our present understanding of the function of these synapses is based on studies of synaptic transmission at the nerve-muscle synapse, the junction between a motor neuron and a skeletal muscle fiber. This is the site where synaptic transmission was first studied and remains best understood. Moreover, the nerve-muscle synapse is the site of a number of inherited and acquired neurological diseases. Therefore, before we examine the complexities of synapses in the central nervous system, we will examine the basic features of chemical synaptic transmission at the nerve-muscle synapse.
The nerve-muscle synapse is an ideal site for studying chemical signaling because it is relatively simple and accessible to experimentation. The muscle cell is large enough to accommodate the two or more micro-electrodes needed to make electrical measurements. Also the muscle cell normally receives signals from just one presynaptic axon, in contrast to the convergent connections on central nerve cells. Most importantly, chemical signaling at the nerve-muscle synapse involves a relatively simple mechanism: Release of neurotransmitter from the presynaptic nerve directly opens a single type of ion channel in the postsynaptic membrane.
The Neuromuscular Junction Is a Well-Studied Example of Directly Gated Synaptic Transmission
The motor neuron innervates the muscle at a specialized region of the muscle membrane called the end-plate, where the motor axon loses its myelin sheath and splits into several fine branches. The ends of the fine branches form multiple expansions or varicosities, called synaptic boutons, from which the motor neuron releases its transmitter. Each bouton is positioned over a specialized region of the muscle membrane containing deep depressions, or junctional folds, which contain the transmitter receptors (Figure 9–1). The transmitter released by the motor axon terminal is acetylcholine (ACh) and the receptor on the muscle membrane is the nicotinic type of ACh receptor.1
Figure 9-1 The neuromuscular junction is an ideal site for studying chemical synaptic signaling. At the muscle the motor axon ramifies into several fine branches approximately 2 µm thick. Each branch forms multiple swellings called synaptic boutons, which are covered by a thin layer of Schwann cells. The boutons lie over a specialized region of the muscle fiber membrane, the end-plate, and are separated from the muscle membrane by a 100-nm synaptic cleft. Each bouton contains mitochondria and synaptic vesicles clustered around active zones, where the neurotransmitter acetylcholine (ACh) is released. Immediately under each bouton in the end-plate are several junctional folds, the crests of which contain a high density of ACh receptors.
The muscle fiber and nerve terminal are covered by a layer of connective tissue, the basal lamina, consisting of collagen and glycoproteins. Unlike the cell membrane, the basal lamina is freely permeable to ions and small organic compounds, including the transmitter. Both the presynaptic terminal and the muscle fiber secrete proteins into the basal lamina, including the enzyme acetylcholinesterase, which inactivates the ACh released from the presynaptic terminal by breaking it down into acetate and choline. The basal lamina also organizes the synapse by aligning the presynaptic boutons with the postsynaptic junctional folds. (Adapted, with permission, from McMahan and Kuffler 1971.)
The presynaptic and postsynaptic membranes are separated by a synaptic cleft approximately 100 nm wide. Within the cleft is a basal lamina (or basement membrane) composed of collagen and other extracellular matrix proteins. The enzyme acetylcholinesterase, which rapidly hydrolyzes ACh, is anchored to the collagen fibrils of the basal laminae. In the muscle cell, in the region below the crest of the junctional fold and extending into the fold, the membrane is rich in voltage-gated Na+ channels (Figure 9–1).
Each synaptic bouton contains all the machinery required to release neurotransmitter. This includes the synaptic vesicles, which contain the transmitter ACh, and the active zones, regions of the membrane specialized for release of transmitter, where the synaptic vesicles are clustered (Figure 9–1). In addition, each active zone contains voltage-gated Ca2+ channels that permit Ca2+ to enter the terminal with each action potential (see Figure 9-1). This influx of Ca2+ triggers the fusion of the synaptic vesicles with the plasma membrane at the active zones, releasing the contents of the synaptic vesicle into the synaptic cleft by the process of exocytosis (see Chapter 12). Every active zone in the presynaptic membrane is positioned opposite a junctional fold in the postsynaptic cell. At the crest of each fold the receptors for ACh are clustered in a lattice, with a density of approximately 10,000 receptors per µm2 (Figure 9–2). The nicotinic ACh receptor is a ligand-gated channel: It is an integral membrane protein that both binds ACh and forms an ion channel (Figure 9–3).
Figure 9-2 Acetylcholine receptors in the vertebrate neuromuscular junction are concentrated at the top one-third of the junctional folds. This receptor-rich region is characterized by an increased density of the postjunctional membrane (arrow). The autoradiograph shown here was made by first incubating the membrane with radiolabeled α-bungarotoxin, which binds to the ACh receptor (black grains). Radioactive decay results in the emittance of a particle that causes overlaid silver grains to become fixed along its trajectory (black grains). Magnification ×18,000. (Reproduced, with permission, from Salpeter 1987.)
Figure 9-3 Low-resolution structure of the acetylcholine (ACh) receptor-channel. These reconstructed electron microscope images were obtained by computer processing of negatively stained images of ACh receptors in the fish Torpedo californica. The resolution is fine enough to see overall structures but too coarse to resolve individual atoms.
A. View looking down on the receptor from the extracellular space. The overall diameter of the receptor and its channel is approximately 8.5 nm. (Reproduced, with permission, from Brisson and Unwin 1985.)
B. Side view of the receptor in the lipid bilayer. The pore is wide at the external and internal surfaces of the membrane but narrows considerably within the lipid bilayer. The channel extends some distance into the extracellular space. A molecule of ACh enters a crevice in the wall of the receptor. (Adapted, with permission, from Karlin 2002; Miyazawa et al. 1999.)
The Motor Neuron Excites the Muscle by Opening Ligand-Gated Ion Channels at the End-Plate
The release of transmitter from the motor nerve terminal opens ACh receptor-channels in the muscle membrane at the end-plate, and this action rapidly depolarizes the membrane. The resulting excitatory postsynaptic potential (EPSP), also called the end-plate potential at the nerve-muscle synapse, is very large; stimulation of a single motor cell produces a synaptic potential of approximately 70 mV.
This change in membrane potential usually is large enough to rapidly activate the voltage-gated Na+ channels in the junctional folds, converting the end-plate potential into an action potential, which then propagates along the muscle fiber. In contrast, in the central nervous system most presynaptic neurons produce postsynaptic potentials less than 1 mV in amplitude. As a result, input from many presynaptic neurons is needed to generate an action potential in most neurons.
The End-Plate Potential Is Produced by Ionic Current Through Acetylcholine Receptor-Channels
The end-plate potential was first studied in detail in the 1950s by Paul Fatt and Bernard Katz using intracellular voltage recordings. Fatt and Katz were able to isolate the end-plate potential by applying the drug curare2 to reduce the amplitude of the postsynaptic potential below the threshold for the action potential (Figure 9–4). They found that the EPSP in muscle cells was largest at the end-plate and decreased progressively with distance (Figure 9–5).
Figure 9-4 The end-plate potential can be isolated pharmacologically for study.
A. Under normal circumstances stimulation of the motor axon produces an action potential in a skeletal muscle cell. The dashed line shows the inferred time course of the end-plate potential that triggers the action potential.
B. Curare blocks the binding of acetylcholine (ACh) to its receptor and so prevents the end-plate potential from reaching the threshold for an action potential (dashed line). In this way the currents and channels that contribute to the end-plate potential, which are different from those producing an action potential, can be studied. The end-plate potential shown here was recorded in the presence of a low concentration of curare, which blocks only a fraction of the ACh receptors. The values for the resting potential (-90 mV), end-plate potential, and action potential in these intracellular recordings are typical of a vertebrate skeletal muscle.
Figure 9-5 The end-plate potential decreases with distance as it passively propagates away from the end-plate. (Adapted, with permission, from Miles 1969.)
A. The amplitude of the postsynaptic potential decreases and the time course of the potential slows with distance from the site of initiation in the end-plate.
B. The decay results from leakiness of the muscle fiber membrane. Because charge must flow in a complete circuit, the inward synaptic current at the end-plate gives rise to a return outward current through resting channels and across the membrane (the capacitor). This return outward flow of positive charge depolarizes the membrane. Because current leaks out all along the membrane, the outward current decreases with distance from the end-plate.
From this, Fatt and Katz concluded that the end-plate potential is generated by an inward ionic current that is confined to the end-plate and then spreads passively away. (Remember, an inward current corresponds to an influx of positive charge, which depolarizes the inside of the membrane.) Inward current is confined to the end-plate because the ACh receptor-channels are concentrated there, opposite the presynaptic terminal from which transmitter is released.
The end-plate potential rises rapidly but decays more slowly. The rapid rise is caused by the sudden release into the synaptic cleft of ACh, which diffuses rapidly to the receptors at the end-plate. (Not all the ACh reaches receptors, however, because ACh is quickly removed from the synaptic cleft by hydrolysis by acetylcholinesterase and diffusion.)
The current that generates the end-plate potential was first studied in voltage-clamp experiments (see Box 7-1). These studies revealed that the end-plate current rises and decays more rapidly than the resultant end-plate potential (Figure 9–6). The time course of the end-plate current is directly determined by the rapid opening and closing of the ACh receptor-channels. Because it takes time for an ionic current to charge or discharge the muscle membrane capacitance, and thus alter the membrane voltage, the EPSP lags behind the synaptic current (see Figure 6-15 and the Postscript at the end of this chapter).
Figure 9-6 The end-plate current increases and decays more rapidly than the end-plate potential.
A. The membrane at the end-plate is voltage-clamped by inserting two microelectrodes into the muscle at the end-plate. One electrode measures membrane potential (Vm) and the second passes current (Im). Both electrodes are connected to a negative feedback amplifier, which ensures that sufficient current (Im) is delivered so that Vm will remain clamped at the command potential Vc. The synaptic current evoked by stimulating the motor nerve can then be measured at constant Vm, for example -90 mV (see Box 7-1).
B. The end-plate potential (measured when Vm is not clamped) changes relatively slowly and lags behind the more rapid inward synaptic current (measured under voltage-clamp conditions). This is because synaptic current must first alter the charge on the membrane capacitance of the muscle before the muscle membrane is depolarized.
The Ion Channel at the End-Plate Is Permeable to Both Sodium and Potassium
Why does the opening of the ACh receptor-channels lead to an inward current that produces the depolarizing end-plate potential? And which ions move through the ACh-gated channels to produce this inward current? One important means of identifying the ion (or ions) responsible for the synaptic current is to measure the value of the chemical driving force (the chemical battery) propelling ions through the channel. Remember, the current through a set of membrane channels is given by the product of the membrane conductance and the electrochemical driving force on the ions conducted through the channels (see Chapter 6). The end-plate current that underlies the EPSP is determined by:
where IEPSP is the end-plate current, gEPSP is the conductance of the ACh receptor-channels, Vm is the membrane potential, and EEPSP is the chemical driving force or battery generated by the transmembrane concentration gradients of the ions conducted through the channels.
The fact that current through the end-plate is inward at the normal resting potential of a muscle cell (-90 mV) indicates that there is an inward (negative) electrochemical driving force on the ions that carry current through the ACh receptor-channels at this potential. Thus, EEPSP must be positive to -90 mV.
The value of EEPSP in Equation 9–1 can be determined by altering Vm in a voltage-clamp experiment and determining its effect on IEPSP. Depolarizing the membrane reduces the net inward electrochemical driving force, thus decreasing the magnitude of the inward end-plate current. If Vm is set equal to EEPSP, there will be no net current through the end-plate channels because the electrical driving force (Vm) will exactly balance the chemical driving force (EEPSP). The potential at which the net ionic current is zero is the reversal potential for current through the synaptic channels; by determining the reversal potential we can experimentally measure the value of EEPSP. If Vm is made more positive than EEPSP, there will be a net outward driving force. In that case stimulation of the motor nerve leads to an outward ionic current (by opening the ACh receptor-channels) that hyperpolarizes the membrane.
If an influx of Na+ were solely responsible for the end-plate potential, the reversal potential for the excitatory postsynaptic potential would be the same as the equilibrium potential for Na+. Thus, if Vm is experimentally altered from -100 to +55 mV, the end-plate current should diminish progressively because the electrochemical driving force on
is reduced. At +55 mV the inward current should be abolished, and at potentials more positive than +55 mV the end-plate current should reverse in direction and become outward.
Instead, experiments at the end-plate showed that as Vm is reduced, the inward current rapidly becomes smaller and is abolished at 0 mV. At values more positive than 0 mV the end-plate current reverses direction and becomes outward (Figure 9–7). This reversal potential is not equal to the equilibrium potential for Na+ or any of the other major cations or anions. In fact, this chemical potential is produced not by a single ion species but by a combination of ions: The ligand-gated channels at the end-plate are almost equally permeable to both major cations, Na+, and K+. Thus, during the end-plate potential Na+ flows into the cell and K+ flows out. The reversal potential is at 0 mV because this is a weighted average of the equilibrium potentials for Na+, and K+ (Box 9-1). At the reversal potential the influx of Na+ is balanced by an equal efflux of K+ (Figure 9–7).
Figure 9-7 The end-plate potential is produced by the simultaneous flow of Na+, and K+ through the same receptor-channels. The ionic currents responsible for the end-plate potential can be determined by measuring the reversal potential of the end-plate current using a voltage clamp.
A. In the hypothetical case in which Na+ flux alone is responsible for the end-plate current, the reversal potential would occur at +55 mV, the equilibrium potential for Na+ (ENa). The arrow at the right of each current record reflects the magnitude of the net Na+ flux at that membrane potential. (Down arrows = inward; up arrows = outward).
B. In the actual case in which the ACh receptor-channel is permeable to both Na+, and K+, experimental results show that the end-plate current reverses at 0 mV because the ion channel allows Na+, and K+ to move into and out of the cell simultaneously (see Box 9-1). The net current is the sum of the Na+, and K+ fluxes through the end-plate channels. At the reversal potential (EEPSP) the inward Na+ flux is balanced by an outward K+ flux so that no net charge flows.
Why are the ACh receptor-channels at the end-plate not selective for a single ion species like the voltage-gated Na+ or K+ channels? This is because the diameter of the pore of the ACh receptor-channel is substantially larger than that of the voltage-gated channels. Electrophysiological measurements suggest that it may be up to 0.8 nm in diameter, an estimate based on the size of the largest organic cation that can permeate the channel. For example, the permeant cation tetramethylammonium (TMA) is approximately 0.6 nm in diameter. In contrast, the voltage-gated Na+ channel is only permeant to organic cations that are smaller than 0.5 × 0.3 nm in cross section, and voltage-gated K+ channels will only conduct ions less than 0.3 nm in diameter.
The relatively large diameter of the ACh receptor pore is thought to provide a water-filled environment that allows cations to diffuse through the channel relatively unimpeded, much as they would in free solution. This explains why the pore does not discriminate between Na+, and K+. It also explains why even divalent cations, such as Ca2+, can permeate the channel. Anions are excluded, however, by the presence of fixed negative charges in the channel, as described later in this chapter.
The Current Through Single Acetylcholine Receptor-Channels Can Be Measured Using the Patch Clamp
The synaptic current at the end-plate is generated by a couple of hundred thousand channels. Recordings of the current through single ACh receptor-channels, using the patch-clamp technique (see Box 5-1), have provided us with insight into the molecular events underlying the end-plate potential.
Individual Receptor-Channels Conduct All-or-None Unitary Currents
The first successful recordings of single ACh receptor-channel currents from skeletal muscle cells, by Erwin Neher and Bert Sakmann in 1976, showed that the opening of an individual channel generates a very small rectangular step of ionic current (Figure 9–8). At a given resting potential a channel will always generate the same-size current pulse. At -90 mV the current steps are approximately -2.7 pA in amplitude. Although this is a very small current, it corresponds to a flow of approximately 17 million ions per second!
Figure 9-8 Individual ACh receptor-channels conduct an all-or-none elementary current.
A. The patch-clamp technique is used to record currents from single ACh receptor-channels. The patch electrode is filled with salt solution that contains a low concentration of ACh and is then brought into close contact with the surface of the muscle membrane (see Box 5-1). The patch may contain one or many receptor-channels.
B. Single-channel currents from a patch of frog muscle membrane with a resting potential of -90 mV were recorded in the presence of 100 nM ACh. (1) The opening of a channel results in an influx of positive charge (recorded as a downward current step). The patch contained a large number of ACh receptor-channels so that successive openings in the record probably arise from different channels. (2) A histogram plotting the amplitudes of these rectangular pulses (in pA) versus the number of observed pulses with the given amplitude. The histogram has a single peak, indicating that the patch of membrane contains only a single type of active channel with an elementary current that varies randomly around a mean of -2.7 pA (1 pA = 10-12 A). This mean, the elementary current, represents an elementary conductance of 30 pS. (Reproduced, with permission, from B. Sakmann.)
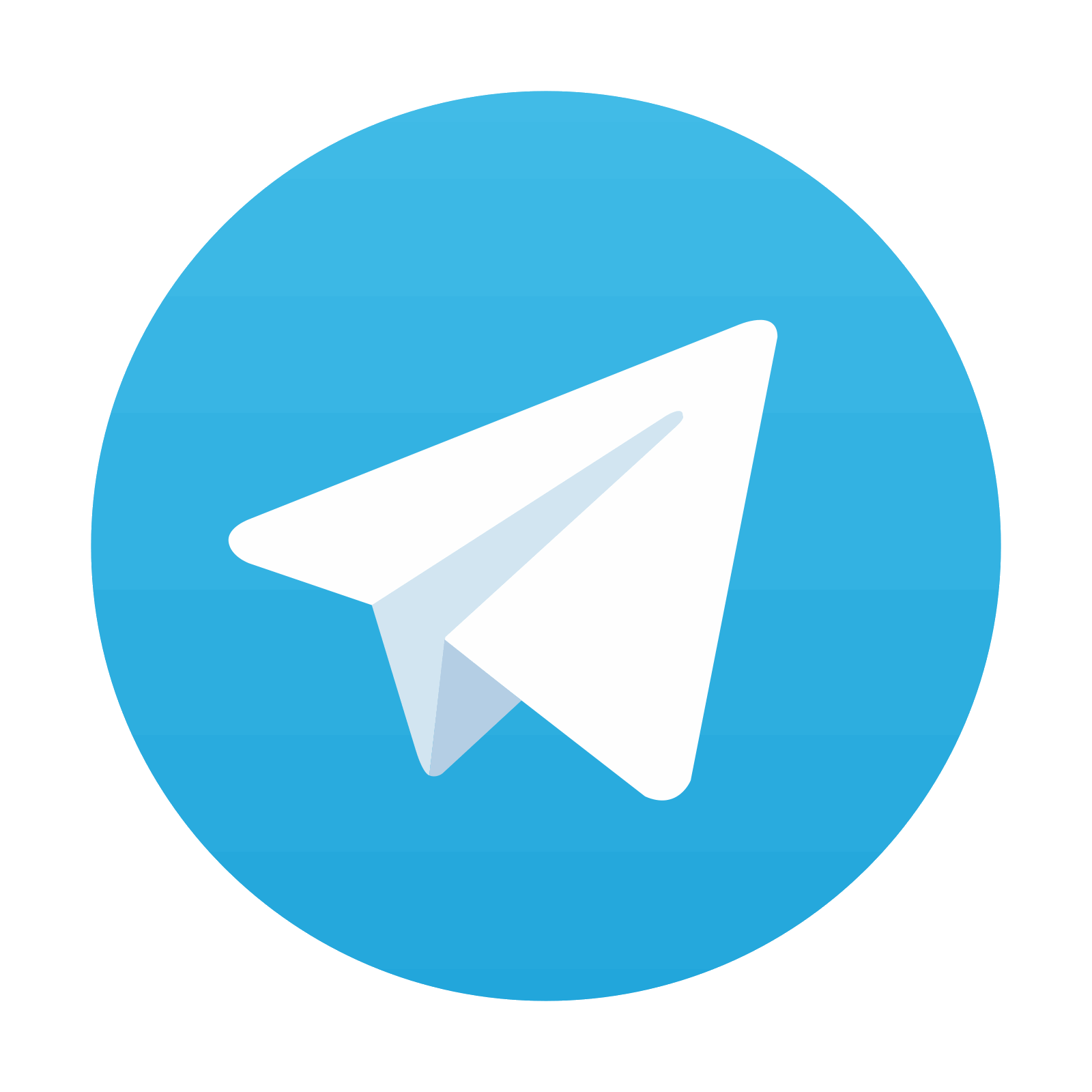
Stay updated, free articles. Join our Telegram channel
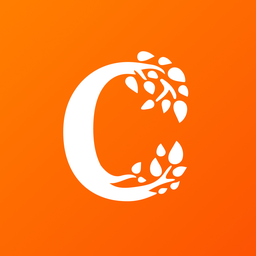
Full access? Get Clinical Tree
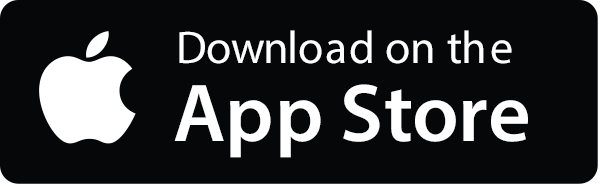
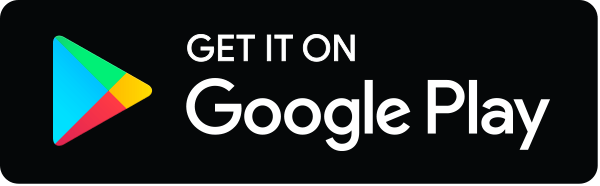