CHAPTER 3
Signaling in the Nervous System
Along with muscle cells, neurons are unique in that they are excitable; that is, they respond to stimuli by generating electrical impulses. Electrical responses of neurons (modifications of the electrical potential across their membranes) may be local (restricted to the place that received the stimulus) or propagated (may travel through the neuron and its axon). Propagated electrical impulses are termed action potentials. Neurons communicate with each other at synapses by a process called synaptic transmission.
MEMBRANE POTENTIAL
The membranes of cells, including nerve cells, are structured so that a difference in electrical potential exists between the inside (negative) and the outside (positive). This results in a resting potential across the cell membrane, which is normally about –70 mV.
The electrical potential across the neuronal cell membrane is the result of its selective permeability to certain charged ions. Cell membranes are highly permeable to most inorganic ions, but they are almost impermeable to proteins and many other organic ions. The difference (gradient) in ion composition inside and outside the cell membrane is maintained by ion pumps in the membrane, which maintain a nearly constant concentration of inorganic ions within the cell (Fig 3–1 and Table 3–1). The pump that maintains Na+ and K+ gradients across the membrane is Na, K-ATPase; this specialized protein molecule extrudes a+ from the intracellular compartment, moving it to the extracellular space, and imports K+ from the extracellular space, carrying it across the membrane into the cell. In carrying out this essential activity, the pump consumes adenosine triphosphate (ATP).
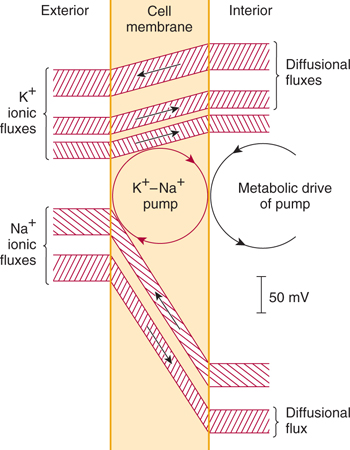
FIGURE 3–1 Na+ and K+ flux through the resting nerve cell membrane. Notice that the Na+/K+ pump (Na+/K+-ATPase) tends to extrude Na+ from the interior of the cell, but it carries K+ ions inward. (Reproduced, with permission, from Eccles JC: The Physiology of Nerve Cells. Johns Hopkins University Press, 1957.)
TABLE 3–1 Concentration of Some Ions Inside and Outside Mammalian Spinal Motor Neurons.
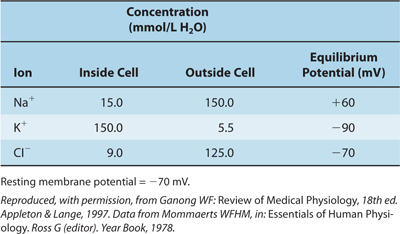
Two types of passive forces maintain an equilibrium of Na+ and K+ across the membrane: A chemical force tends to move Na+ inward and K+ outward, from the compartment containing high concentration to the compartment containing low concentration, and an electrical force (the membrane potential) tends to move Na+ and K+ inward. When the chemical and electrical forces are equally strong, an equilibrium potential exists.
For an idealized membrane that is permeable to only K+, the Nernst equation, which describes the relationship between these forces, is used to calculate the magnitude of the equilibrium potential (ie, the membrane potential at which equilibrium exists). Normally, there is a much higher concentration of K+ inside the cell ([K+]i) than outside the cell ([K+]o) (see Table 3–1). The Nernst equation, which would be used to determine membrane potential across a membrane permeable only to K+ ions, is as follows:
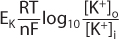
where
At physiologic temperatures
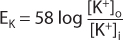
The equilibrium potential (ENa) for sodium can be found by substituting [Na+]i and [Na+]o in the Nernst equation; this potential would be found across a membrane that was permeable only to sodium. In reality, most cell membranes are not perfectly selective; that is, they are permeable to several ionic species. For these membranes, potential is the weighted average of the equilibrium potentials for each permeable ion, with the contribution for each ion weighted to reflect its contribution to total membrane permeability. This is described mathematically, for a membrane that is permeable to Na+ and K+, by the Goldman-Hodgkin-Katz equation (also known as the constant field equation):

where
In the membrane of resting neurons, K+ permeability is much higher (~20-fold) than Na+ permeability; that is, the PK–PNa ratio is approximately 20:1. Thus, when a neuron is inactive (resting), the Goldman-Hodgkin-Katz equation is dominated by K+ permeability so that membrane potential is close to the equilibrium potential for K (EK). This accounts for the resting potential of approximately –70 mV.
GENERATOR POTENTIALS
The generator (receptor) potential is a local, nonpropagated response that occurs in some sensory receptors (eg, muscle stretch receptors and pacinian corpuscles, which are touch-pressure receptors) where mechanical energy is converted into electric signals. The generator potential is produced in a small area of the sensory cell: the nonmyelinated nerve terminal. Most generator potentials are depolarizations, in which membrane potential becomes less negative. In contrast to action potentials (see the next section), which are all-or-none responses, generator potentials are graded (the larger the stimulus [stretch or pressure], the larger the depolarization) and additive (two small stimuli, close together in time, produce a generator potential larger than that made by a single small stimulus). Further increase in stimulation results in larger generator potentials (Fig 3–2). When the magnitude of the generator potential increases to about 10 mV, a propagated action potential (impulse) is generated in the sensory nerve.
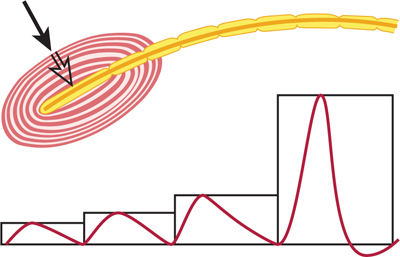
FIGURE 3–2 Demonstration of a generator potential in a pacinian corpuscle. The electrical responses to a pressure (black arrow) of 1×, 2×, 3×, and 4× are shown. The strongest stimulus produced an action potential in the sensory nerve, originating in the center of the corpuscle (open arrow).
ACTION POTENTIALS
Neurons communicate by producing electrical impulses called action potentials. Action potentials are self-regenerative electrical signals that tend to propagate throughout a neuron and along its axon. The action potential is a depolarization of about 100 mV (a large signal for a neuron). The action potential is all or none. Its size is constant for each neuron.
Neurons can generate action potentials because they contain specialized molecules, called sodium channels, that respond to depolarization by opening (activating). When this occurs, the relative permeability of the membrane to Na+ increases, and the membrane moves closer to the equilibrium potential for Na+, as predicted by the Goldman-Hodgkin Katz equation, thus causing further depolarization. When a depolarization (from a generator potential, synaptic potential, or oncoming action potential) impinges on a neuronal membrane, sodium channels activate and, as a result, the membrane begins to further depolarize. This action tends to activate still other sodium channels, which also open and cause depolarization. If a sufficient number of sodium channels are activated, there is a depolarization of about 15 mV, and threshold is reached so that the rate of depolarization increases sharply to produce an action potential (Fig 3–3). Thus, the membrane generates an explosive, all-or-none action potential. As the impulse passes, repolarization occurs rapidly at first and then more slowly. Membrane potential thus returns to resting potential. The action potential tends to last for a few milliseconds.
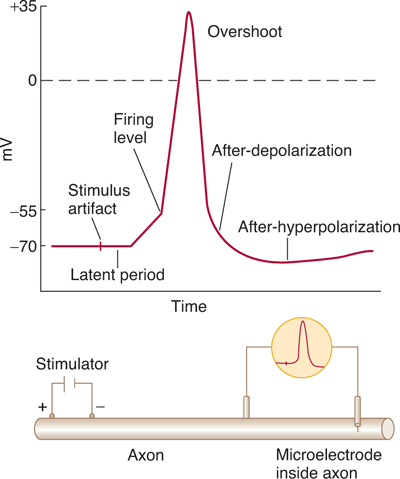
FIGURE 3–3 Action potential (“spike potential”) recorded with one electrode inside cell. In the resting state, the membrane potential (resting potential) is about –70 mV. When the axon is stimulated, there is a small depolarization. If this depolarization reaches the firing level (threshold), there is an all-or-none depolarization (action potential). The action potential approaches ENa and overshoots the 0-mV level. The action potential ends when the axon repolarizes, again settling at resting potential. (Reproduced, with permission, from Ganong WF: Review of Medical Physiology, 22nd ed. McGraw-Hill, 2005.)
In some fibers, membrane potential becomes transiently hyperpolarized (the after-hyperpolarization) as a result of the opening of the K+ channels, which tends to drive the membrane toward EK. In the wake of an action potential, there is a refractory period of decreased excitability. This period has two phases: the initial absolute refractory period, during which another action potential cannot be generated, and the relative refractory period (lasting up to a few milliseconds), during which a second action potential can be generated but conduction velocity is decreased and threshold increased. The refractory period limits the ability of the axon to conduct high-frequency trains of action potentials.
THE NERVE CELL MEMBRANE CONTAINS ION CHANNELS
Voltage-sensitive ion channels are specialized protein molecules that span the cell membrane. These doughnut-shaped molecules contain a pore that acts as a tunnel, permitting specific ions (eg, Na+ or K+), but not other ions, to permeate. The channel also possesses a voltage sensor, which, in response to changes in potential across the membrane, either opens (activates) or closes (inactivates) the channel.
The neuronal membrane has the ability to generate impulses because it contains voltage-sensitive Na+ channels, which are selectively permeable to Na+ and tend to open when the membrane is depolarized. Because these channels open in response to depolarization, and because by opening they drive the membrane closer to Na+ equilibrium potential (ENa), they tend to further depolarize the membrane (Fig 3–4). If a sufficient number of these channels are opened, there is an explosive, all-or-none response, termed the action potential (see Fig 3–3). The degree of depolarization necessary to elicit the action potential is called the threshold.
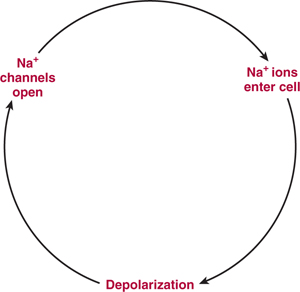
FIGURE 3–4 Ionic basis for the depolarization underlying the action potential. Voltage-sensitive Na+ channels open when the membrane is depolarized. This action results in increased Na+ permeability of the membrane, causing further depolarization and the opening of still other Na+ channels. When a sufficient number of Na+ channels have opened, the membrane generates an explosive, all-or-none depolarization—the action potential.
Other voltage-sensitive ion channels (voltage-sensitive K+ channels) open (usually more slowly than Na+ channels) in response to depolarization and are selectively permeable to K+. When these channels open, the membrane potential is driven toward the K+ equilibrium potential (EK), leading to hyperpolarization.
THE EFFECTS OF MYELINATION
Myelin is present around some axons within the peripheral nervous system (PNS) (where it is produced by Schwann cells) and within the central nervous system (CNS) (where it is produced by oligodendrocytes). Myelination has profound effects on the conduction of action potentials along the axon.
Nonmyelinated axons, in the mammalian PNS and CNS, generally have a small diameter (less than 1 μm in the PNS and less than 0.2 μm in the CNS). The action potential travels in a continuous manner along these axons because of a relatively uniform distribution of voltage-sensitive Na+ and K+ channels. As the action potential invades a given region of the axon, it depolarizes the region in front of it, so that the impulse crawls slowly and continuously along the entire length of the axon (Fig 3–5). In nonmyelinated axons, activation of Na+ channels accounts for the depolarization phase of the action potential, and activation of K+ channels produces repolarization.
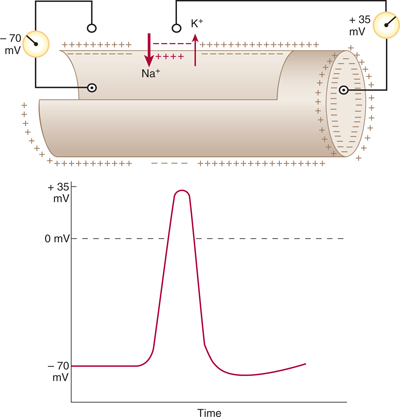
FIGURE 3–5 Conduction of the nerve impulse through a nonmyelinated nerve fiber. In the resting axon, there is a difference of –70 mV between the interior of the axon and the outer surface of its membrane (resting potential). During the conduction of an action potential, Na+ passes into the axon interior and subsequently K+ migrates in the opposite direction. In consequence, the membrane polarity changes (the membrane becomes relatively positive on its inner surface), and the resting potential is replaced by an action potential (+35 mV here). (Reproduced, with permission, from Junqueira LC, Carneiro J, Kelley RO: Basic Histology, 7th ed. Appleton & Lange, 1992.)
Myelinated axons, in contrast, are covered by myelin sheaths. The myelin has a high electrical resistance and low capacitance, permitting it to act as an insulator. The myelin sheath is not continuous along the entire length of the axon. On the contrary, it is periodically interrupted by small gaps (approximately 1 μm long), called the nodes of Ranvier, where the axon is exposed. In mammalian myelinated fibers, the voltage-sensitive Na+ and K+ channels are not distributed uniformly. Na+ channels are clustered in high density (about 1000/pμ2) in the axon membrane at the node of Ranvier, but are sparse in the internodal axon membrane, under the myelin. K+ channels, on the other hand, tend to be localized in the “internodal” and “paranodal” axon membrane, that is, the axon membrane covered by the myelin (Fig 3–6).
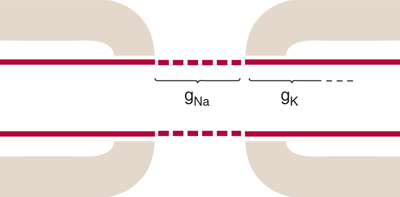
FIGURE 3–6 Na+ and K+ channel distributions in myelinated axons are not uniform. Na+ channels (gNa) are clustered in high density in the axon membrane at the node of Ranvier, where they are available to produce the depolarization needed for the action potential. K+ channels (gK), on the other hand, are located largely in the internodal axon membrane under the myelin, so that they are masked. (Reproduced with permission from Waxman SG: Membranes, myelin and the pathophysiology of multiple sclerosis, N Engl J Med Jun 24;306(25):1529–33, 1982.)
Because the current flow through the insulating myelin is very small and physiologically negligible, the action potential in myelinated axons jumps from one node to the next in a mode of conduction that has been termed saltatory (Fig 3–7). There are several important consequences to this saltatory mode of conduction in myelinated fibers. First, the energy requirement for impulse conduction is lower in myelinated fibers; therefore, the metabolic cost of conduction is lower. Second, myelination results in an increased conduction velocity. Figure 3–8 shows conduction velocity as a function of diameter for nonmyelinated and myelinated axons. For nonmyelinated axons, conduction velocity is proportional to (diameter)1/2. In contrast, conduction velocity in myelinated axons increases linearly with diameter. A myelinated axon can conduct impulses at a much higher conduction velocity than a nonmyelinated axon of the same size. To conduct as rapidly as a 10-μm myelinated fiber, a nonmyelinated axon would need a diameter of more than 100 μm. By increasing the conduction velocity, myelination reduces the time it takes for impulses to travel from one region to another, thus reducing the time needed for reflex activities and permitting the brain to operate as a high-speed computer.
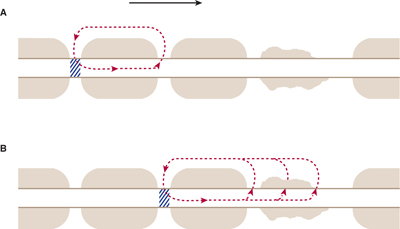
FIGURE 3–7 A: Saltatory conduction in a myelinated axon. The myelin functions as an insulator because of its high resistance and low capacitance. Thus, when the action potential (cross-hatching) is at a given node of Ranvier, the majority of the electrical current is shunted to the next node (along the pathway shown by the broken arrow). Conduction of the action potential proceeds in a discontinuous manner, jumping from node to node with a high conduction velocity. B: In demyelinated axons there is loss of current through the damaged myelin. As a result, it either takes longer to reach threshold and conduction velocity is reduced, or threshold is not reached and the action potential fails to propagate. (Reproduced, with permission, from Waxman SG: Membranes, myelin and the pathophysiology of multiple sclerosis. N Engl J Med 1982;306:1529.)
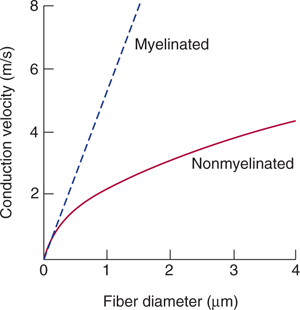
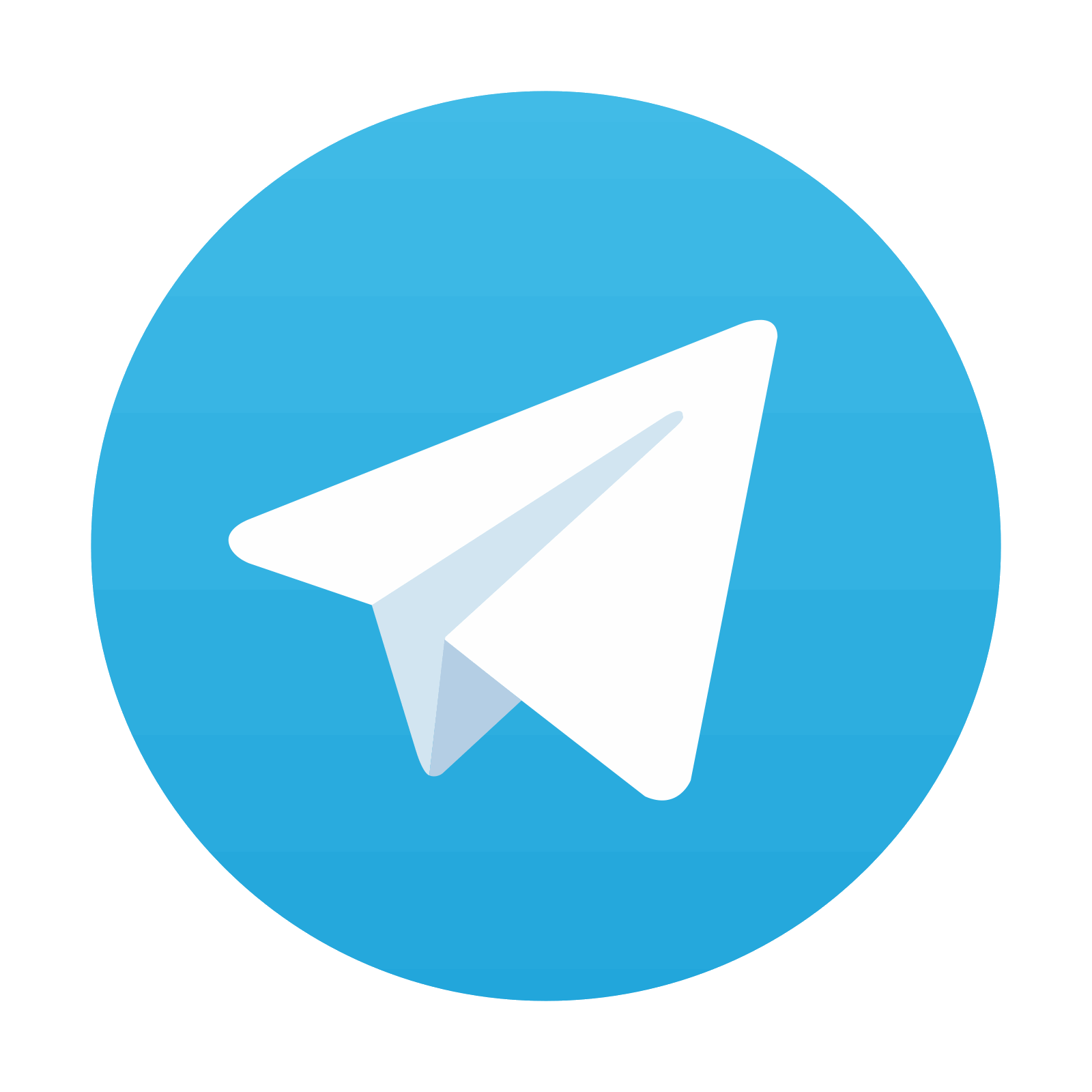
Stay updated, free articles. Join our Telegram channel
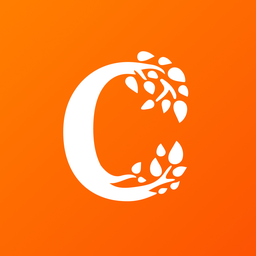
Full access? Get Clinical Tree
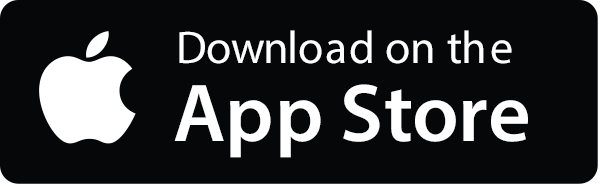
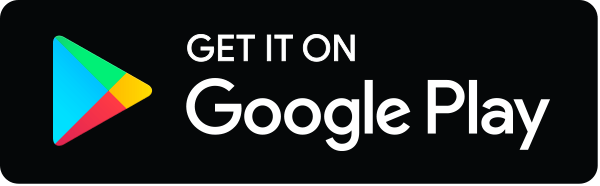