and Michele Salanova1
(1)
Vegetative Anatomy, Charité Universitätsmedizin Berlin, Berlin, Germany
Abstract
This chapter is an update of the current view of the general structure and function of the human skeletal muscle system with its functional muscle groups (e.g., antigravity muscles) with special emphasis on the almost forgotten muscular reinforcement structures (fascia and entheses) and on the myocellular force-producing little power chambers inside skeletal muscle fibers known as sarcomeres. Skeletal muscle is well adapted to gravitational loading (1G) on Earth but is highly challenged to microgravity unloading in Space (μG, zero-G). In particular, we found that nitric oxide (NO) signaling and nitrosative stress management (via protein S-nitrosylation) in human skeletal muscle provide reliable signatures to assess efficacy of physical countermeasures. We use high-resolution confocal laser microscopy for the precise immunohistochemical biomarker detection at the cellular and subcellular level combined with quantitative biochemical methods and current protein mapping by 2-DIGE proteomics to comprehensively document disuse changes in normal healthy muscle and to assess efficacy of different exercise countermeasures against disuse atrophy. Over more than 10 years, our laboratory cooperates with national and international multidisciplinary Space Life Sciences research groups in ground-based experiments (mice) and in several bed rest studies, for example, using resistive vibration exercise (RVE) as a very efficient countermeasure against chronic disuse. Published and preliminary results from two long- and medium-term spaceflight experiments with mice, one on the International Space Station (91 days MDS mission) and another one on a Russian biosatellite on orbit (30 days BION M1 mission), provided new insights to cell signaling changes in mammalian skeletal muscle in real microgravity.
Keywords
Skeletal muscleNitric oxide signalingNitrosative stressGround-based experimentsPhysical countermeasureSpaceflight2.1 Skeletal Muscle Structure and Function
2.1.1 Functional Anatomy of Normal Human Skeletal Muscle
The human skeletal muscle system comprises about 220 specific muscles with various sizes, shapes, locations, and functions in the body. Some are relatively small (<3 cm in length) such as some hand and foot muscles (interossei, lumbricales) used for complex finger/toe movement control (grasping, playing on instruments) or some deep medial column back muscles (rotators) for local segmental spine rotations. Some are even smaller (<3 mm) and used for fine-tuning of discrete movements (Alkner and Tesch 2004) (e.g., the little stapedius controls movements of the last of three middle ear bones) in acoustic sensation and hearing. Others are longer (>40 cm, sartorius, erector spinae), while others are broader and rather powerful and fleshy (quadriceps femoris, latissimus dorsi, adductors) for use in body stabilization (posture, gait) and mobilization (movements, performance). Another functional group is located at deep muscle layers adjacent to bones running closely over one or more joints (single vs. multi-joint muscles) to facilitate local joint movement and stabilization (see Sect. 2.1.4). In general, almost 60 % of the functional skeletal musculature in the healthy human body is used for body stabilization and postural control during stance and body motions in normal everyday life on Earth and is thus termed “antigravity” muscles (Fig. 2.1).
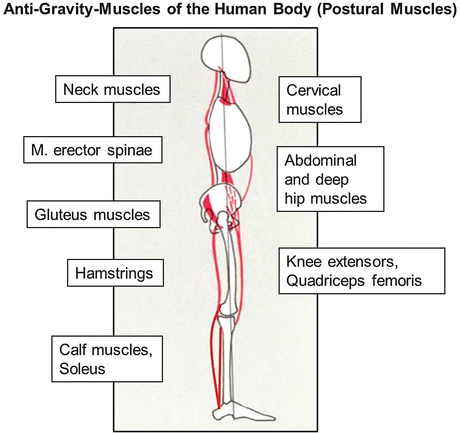
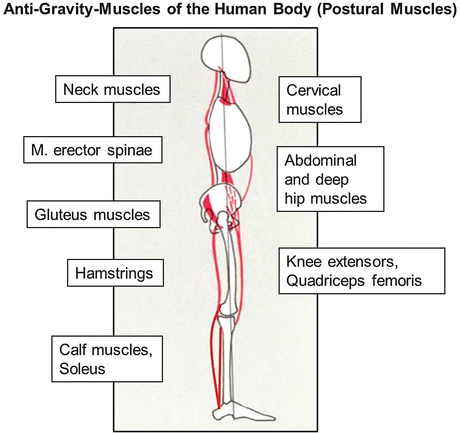
Fig. 2.1
Cartoon showing the key “antigravity” skeletal muscle groups of the human body for postural control of body stabilization, during stance, gait, and other motions/movements on Earth (1G)
Most of the skeletal muscles are usually grouped in the body by anatomic and functional compartments classified according to their body location and function in the trunk or extremities as ventral/dorsal, medial/lateral, and extensor/flexor compartments. For example, the soleus and gastrocnemius belong to the superficial dorsal calf plantar flexors (triceps surae) which insert via the Achilles tendon to the calcaneus bone (Fig. 2.1). The adductor muscles (short, long, and greater adductor, gracilis) belong to the medial thigh compartment (thigh adductors) for hip stabilization and leg adduction. Muscle compartments are located underneath the main body fascia (similar to a whole body stocking or cat suit) separated from the skin above (Schleip et al. 2012). The muscle compartments underneath the main body fascia particularly of the arms and legs resemble long spaces separated by fibrous connective tissue sheets known as fascia sheets or box fascia which usually contain about two or three individual muscles (e.g., the long and short fibularis of the lateral calf compartment) or two-/three-headed single muscles (e.g., biceps/triceps of ventral arm flexors/dorsal arm extensors). Each compartment is comparable to a box with functionally grouped (extensor/flexor/adductor) muscles that receive a common neurovascular supply (nerve and blood vessels) from deep main arteries or peripheral nerves and thus participate in the functional anatomy of human skeletal muscle (Blottner 2013). In most body regions, the fascia layers are part of the soft muscle tissue (Fig. 2.2) and may not be easily palpable, while others are more stiff (i.e., great thoracolumbar fascia) and well palpable on the back superficial lumbar region or at the lateral hip region (iliotibial tract) of the lower limb.
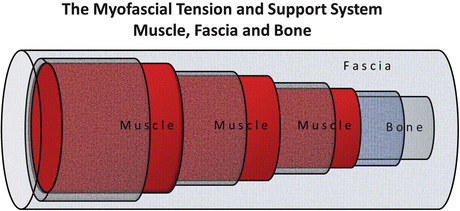
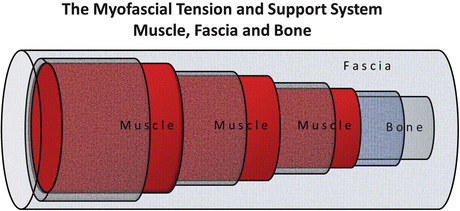
Fig. 2.2
Proposed topographic and biodynamic model for the human myofascial tension and support system comprised of bone with periost (inner semilucent tube), up to three skeletal muscle layers (red) with muscle fascia (lucent tubes), and the general fascia support of the muscle compartments (Fascia, overarching lucent tube). In this model, the bone structures represent force and strain-resistant support (by relative stiffness), the muscle layers represent actively contracting elements (tension and force production), and the various fascial structures represent passive viscoelastic support (tension and force storage) by connective tissue components, collagen fiber networks, and extracellular matrix molecules. The biomechanical properties (biomechanical stress response) of active and passive structures shown here may be affected in periods of disuse (unloading) and aging on Earth and during microgravity adaptation following long-term spaceflight
2.1.2 The Reinforcement Structures: Human Resting Myofascial Tension (HRMT) System and Enthesis Organs (Including Tendons)
The contracting skeletal muscle (shortening of muscle) is the major force-producing component in human movement and performance probably representing one of the major vital expressions of life (e.g., contractile proteins – > muscle – > locomotion = > expression of life). However, a set of other nonmuscular connective tissue structural network always work in cooperation with normal muscle tension, contraction, and relaxation in order to adequately transmit the local biomechanical forces from the individual muscle fibers of a single muscle of a functional muscle group (extensors vs. flexors), for example, via muscle tendons inserted to the jointed bone levers throughout the human body to reinforce muscle contractions during various body motions in normal gravity (1G) on Earth. More recently, the structure and function of the various (almost forgotten) body fascia layers and, in particular, interface structures involved in biomechanical friction termed “enthese organs” (Benjamin et al. 2006) including tendons, myotendinous junctions (muscle-tendon interface), and osseotendinous junctions (tendon-bone interface), the retinacula of the knee and ankle joint, or even the flat tendinous structures known as aponeuroses that attach flat muscles to bone, for example, the great thoracicolumbar fascia of the Latissimus dorsi back muscle, have been reconsidered as key biodynamical support structures of the human body. In addition, the dense regular and mesh-like connective tissue of a body-wide network of compression and tension (i.e., 3D tensegrity model of stiff and elastic structures) received more and more attention in the literature (Schleip et al. 2012). So far, the biomechanical roles of the body fascia under physiological and clinical aspects are only beginning to be reinvestigated as critical elements of an integrative body system termed the human resting myofascial tone and tension (HRMT) system (Masi et al. 2010).
The current view is that an estimated amount of about 10–30 % of total muscle power and force in the human body appears to be more or less stored by functional fascia and entheses related to the skeletal muscle system in normal everyday life or in exercise and sports (Huijing and Baan 2003). An altered structural composition of muscle or body fascia, for example, following longer periods of disuse or in the elderly may likely change the biomechanical properties (fascia may become either more flexible or stiff); however, very little is known about such adaptation mechanisms of the HMRT system in microgravity. As already routinely used in physical and rehabilitation medicine, physiotherapy, or osteopathy in practice, the term “myofascial tension system” should be used in addition to the term musculoskeletal system in the Human Space Life Sciences in order to underline its important structural and functional role for human performance control on Earth that were proposed to be further investigated in future ground-based analogues (bed rest disuse) and in human spaceflight (Lang T et al. Effect of long-duration spaceflight on bone and muscle: Report of the Bone-Muscle Expert Group, Cluster 1, Integrated Systems Physiology, Theseus Project of ESA, European Science Foundation, and European Community’s 7th Framework Program, FP7/2007–2013, unpublished).
In order to stimulate future research on the biomechanical properties of the various support systems of the musculoskeletal system, a comprehensive hypothetical biodynamic model for the human myofascial tension and support system is presented here that is based on functional anatomy and biodynamic properties of skeletal muscle, fascia, and bone and that is also used by the author in his academic teaching for second year medical students of the teaching module “Movement and Exercise” at the Charité Berlin, Germany (Fig. 2.2).
2.1.3 The Little Power Chambers Inside: Sarcomere, Sarcoplasmic Reticulum (SR), and More
A whole skeletal muscle is composed of larger and smaller bundles of skeletal myofibers (secondary and primary fascicles) mostly running throughout the length of a muscle and separated by perimysial connective tissue layers (Fig. 2.3). The secondary fascicles (sarcous fibers) are usually visible with the eye. In the microscope, a single muscle fiber is seen as a spindle-shaped elongated cell tube (about 20 cm in length, thickness comparable to a spiders thread, approx. 100 μm) closely packed with bundles of thin myofibrils composed of much thinner microfilaments (thin actin, thick myosin filaments), with the calcium-dependent regulatory proteins (tropomyosin, troponin) responsible for actin-myosin interaction and the accessory proteins titin (spring-like protein) and nebulin (actomyosin stiffness) responsible for structural integrity, elasticity, and stiffness within the basic force-producing contractile component known as the sarcomere with its typical striated patterns (seen in light and electron microscopy) of A- and I-bands of overlapping actin-myosin microfilaments each anchored to the subtended Z-disks as the microstructural demarcations of a sarcomere (lat. sarcos = flesh) (Fig. 2.4, manuscript in preparation). Numerous sarcomeres (little power chambers) are arranged in series of about 500/mm of a muscle fiber so that the microscopic shortenings of individual sarcomeres add up to the visible muscle contractions and macroscopic changes in muscle length, for example, during body motions. Skeletal muscle fibers contain all the typical cell organelles found in any other nucleated cell type (mitochondria, Golgi apparatus, endoplasmic reticulum, vesicles), but, unlike most single-nucleated cell types of the body, they contain about a hundred of myonuclei for gene transcriptional control of subcellularly partitioned microdomains for normal cell metabolism and adaptation. Muscle fibers also contain a complex and specialized endoplasmic network of tubules known as the sarcoplasmic reticulum (SR) that express a set of calcium release and calcium uptake proteins (ryanodine receptor, SERCA) which actively transport calcium ions from this major calcium store to the sarcosol and vice versa (influx/efflux) to enable normal fiber contraction during excitation. For more details, refer to a current book chapter by Brenner and Maasen (2013) and shown in this chapter (Fig. 2.18).
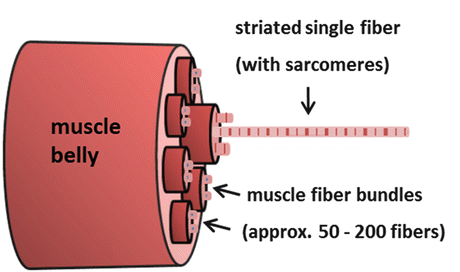
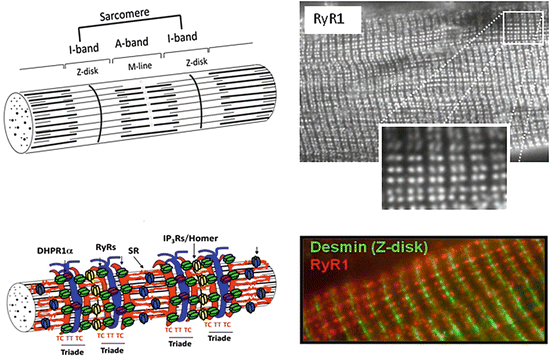
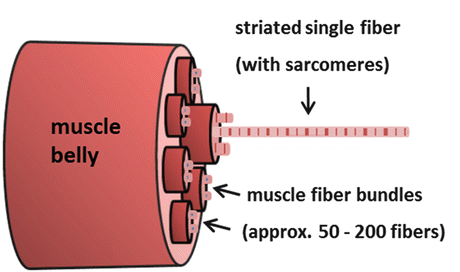
Fig. 2.3
A skeletal muscle (muscle belly) is ensheathed by a fascia and is composed of larger and smaller groups of fiber bundles (secondary and primary fascicles) embedded in loose connective tissue layers (perimysium) with neurovascular supply (capillaries, nerves, fibroblasts). A muscle fiber bundle contains approximately 50–200 striated individual fibers (polynucleated cell tube, sized 100 μm) embedded in a delicate connective tissue layer (endomysium). Each thin fiber is ensheathed by a basal lamina that provides mechanical support with extracellular matrix anchor molecules (laminin, fibronectin). Striated single muscle fibers contain bundles of myofibrils (1 μm) with their sarcomeres (little power chambers, sized 2–4 μm) in series (Fig. 2.4)
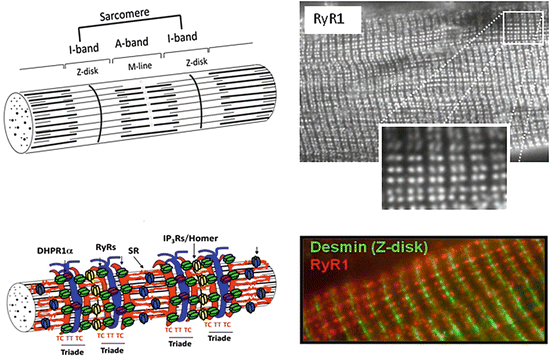
Fig. 2.4
Ultrastructural representation of a contractile sarcomere (serially power chamber) isolated from a myofibrillar bundle (upper panel) and its peri-sarcomeric support elements. As integral serial part of one long myofibril in a muscle fiber, the uncovered sarcomere structure has two adjacent endings (Z-disks) where the thin actin and thick myosin filaments are anchored and show overlapping regions. The main areas of thin actin microfilaments (I-band) and overlapping areas of thin actin and thick myosin microfilaments (A-band) are shown in the upper cartoon without support elements such as tubuli and other functional proteins. For better overview, the accessory sarcomeric filament proteins titin (filamentous spring-like stabilizers that span throughout the sarcomere from the adjacent Z-disks) and nebulin (connectivity functions during actin-myosin interactions) are not shown (lower panel, left). The sarcomeres are surrounded (covered) by tubular networks of the sarcoplasmic reticulum structures (SR) of the longitudinal system (L-tubuli, thin red lines) with terminal cisterns (TC, thick red semicircles) and transverse tubules (TT, thick blue semicircles) which are membrane extensions of the outer membrane into the muscle fiber. The main typical SR-associated calcium channel proteins are shown by four subunit protein icons of various colors, RyR1 (green), SERCA (blue), DHPR (purple), and IP3R/Homer (yellow). Another protein called Homer is located adjacent to Z-disk structures but also at the subsynaptic microdomain (not shown, see Chap. 3.0). Lower panel (right) shows double staining by immunohistochemistry with anti-desmin (Z-disk marker, green) and anti-RyR1 (red) antibodies in two or three adjacent striated rat muscle fibers (Manuscript in preparation)
2.1.4 Muscle Works Against Gravitational Forces on Earth
In order to cushion the gravitational load forces, movements of the human body are biodynamically controlled via muscle by muscle contraction in accordance with a typical pattern known as “closed muscle chains” (e.g., activated in squat down by knee bending). In “open muscle chain” movements, for example, arms or legs are freely moved away from the body axis however against gravitational load but in absence of whole body weight loading (e.g., lifting of the arms or of one leg only during standing or recumbent body position). A more detailed description of the human skeletal muscle system based on topographical and functional muscle compartments is found in a current book chapter elsewhere (Blottner 2013).
In microgravity, movements of the floating human body are mainly performed according to the open muscle chain type due to gravitational unloading. However, gravitational loading can be simulated during inflight exercise by pressing the body with elastic straps fixed to comfortable body harnesses (shoulder and hip) to a fixed point of an exercise device during the training bouts, thus simulating closed muscle chain movements at least under partial resistance loading conditions in spaceflight (see Sect. 2.1.1 and next chapters).
2.1.5 Current Definitions of Human Skeletal Muscle Contraction Types Under Normal Gravity Conditions on Earth (1G)
Dynamic skeletal muscle is attached to relatively stable or stiff bone (point of origin) via more flexible osseotendinous junctions (muscle-tendon-bone) and further runs over joints to their points of insertion on another bone (usually distal to the same joint). The main function of skeletal muscle is contraction (shortening) which brings the two bones (lever arms) closer together to initiate active mode body motions during voluntary movements. This type of a “visible” muscle activity is usually considered by most people as a muscle contraction with force production. In passive mode body motions, however, muscles are passively moved (i.e., they are shortened or slightly stretched) against gravitational load (i.e., passive arm or leg movements without voluntary forces) usually performed without one’s own muscle force, however, with the help of the force of a therapist (Sect. 2.2.1.1). The muscle in action is termed the agonist (e.g., biceps brachii during arm bending), while its counterpart is termed the antagonist (e.g., triceps brachii during arm bending). In this example, active and passive stretching of skeletal muscle is performed alternatingly between agonist and antagonist contractions during normal elbow joint movements.
The basic muscular contractions include isotonic contraction (muscle shortens in length under constant work/tension against gravity, e.g., arm lifting a heavy book from table for reading, maximal force is larger than the gravitational load of an object), isometric contraction (muscle remains the same length under constant work/tension against gravity, e.g., holding a heavy book in front of the body or carrying a heavy bag, the muscle force precisely matches the gravitational load), and auxotonic contraction (simultaneous change in length and force under constant work against gravity, e.g., heavy weight lifters, muscle force becomes higher during lifting motions). In addition, stopped preloaded contractions (e.g., masticatory pressure following teeth occlusion) and afterload contractions (e.g., lifting an object with changed effective lever arms) are composite types of contractions including isotonic, isometric, and auxotonic contractions (Brenner and Maasen 2013).
In muscle exercise, muscular strength (force) is usually increased by resistance (resistive) training which is a combination between concentric (shortening contraction, force generated while shortening occurs sufficient to overcome gravitational load) and eccentric muscle loading (lengthening contraction, force generated while muscle elongates under tension such as during deceleration of an object or lowering an object gently, the force generated becomes more and more insufficient to overcome gravitational load). Eccentric muscle contraction is typically performed during sit-ups, squatting exercises, or arm press-ups (body weight load) or with additional loading using barbells, dumbbells, or elastic straps in normal fitness or extensive strength training protocols. Compared to concentric loading, heavy eccentric loading (muscle building, strength training) has some considerable risk of muscle damage (Faulkner 2003).
2.1.6 Histologic and Molecular Adaptation in Normal, Atrophic, and Gravitational Unloaded Skeletal Muscle
Normal human skeletal muscle consists of various types of muscles with predominantly slow (type I, e.g., soleus) and predominantly fast myofiber-type (type II, e.g., brachioradialis) distribution patterns, and a variable number of muscles with mixed slow/fast fiber types (e.g., vastus lateralis) currently reviewed (Blauww et al. 2013; Schiaffino and Reggiani 2011). The normal human vastus lateralis (Fig. 2.5) of younger males (17–33 years of age, Johnson et al. 1973) consists of a mixture of fast-twitch (type II) and slow-twitch (type I) myofibers in a varying composition (approximately 65 % fast vs. 35 % slow fibers), while the antigravity calf muscle, the soleus, mainly consists of slow-type myofibers (approx. 85 % slow vs. 15 % fast). If isoform-specific MyHC subtype markers are used as detectable immunomarkers during human skeletal muscle adaptation, variable numbers of fast myofiber subtypes (IIa, IIx) are usually found, while the IIb fiber subtypes are only found in a few defined human skeletal muscle species (e.g., extraocular, pharyngeal, laryngeal). The MyHC IIb subtypes are, however, frequently found in rat or mice skeletal muscle (Ciciliot et al. 2013).
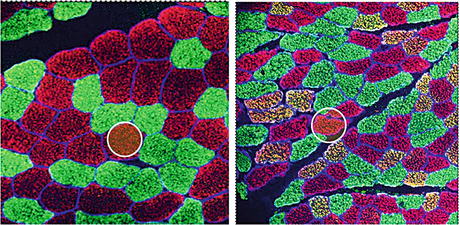
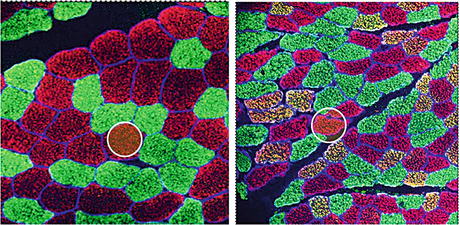
Fig. 2.5
Myofiber-type distribution (mixed 60:40 % fast type 2a,x vs. slow type 1) and cross-sectional area (CSA) size changes in normal (left) versus atrophic (right) human vastus lateralis (VL) after 60 days of disuse in bed rest (BBR-1 study, Charité Berlin). Slow type 1 (green), fast type 2a/x (red), and hybrid (yellow) myofibers expressing both slow and fast myosin heavy chain (s/fMyHC) immunolabels as a sign of disuse-induced fiber slow-to-fast transition. In both images, the myofiber CSA size change is marked in two cross-sectioned myofiber profiles by a white circle (approx. 4,000 mμ2) of identical area
In addition to the size and phenotype distribution changes observed in disuse, the integrity of the outer muscle fiber membrane (sarcolemma) and the subsarcolemmal dystrophin scaffold structures are also compromised in human and animal skeletal muscle disuse. The dystrophin proteins are considered as key proteins of the muscle fiber subsarcolemmal scaffold helping in membrane stabilization and membrane-actin stiffness (Sarkis et al. 2013). In our work, we showed that the subsarcolemmal localized protein dystrophin also serves as a valuable immunohistochemical marker to show altered membrane integrity patterns in normal versus disused human skeletal muscle in cryosections following prolonged disuse in bed rest (Salanova et al. 2014; Blottner et al. in press). The dystrophin immunolabel has been routinely implemented to our immunohistochemical protocols for many years now to clearly identify the outer demarcations of muscle fibers to improve qualitative and quantitative analysis of skeletal muscle fiber structures and biochemical properties on biopsy cryosections in normal versus disused human skeletal muscle as well as in space-related animal studies (Fig. 2.6).
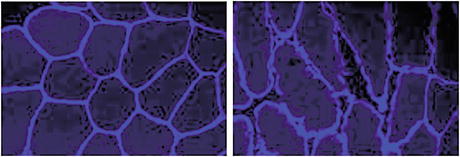
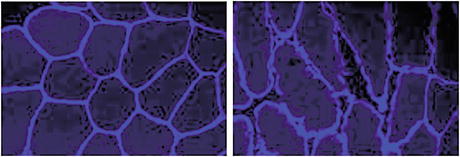
Fig. 2.6
Dystrophin immunohistochemical patterns in cross-sectioned myofibers of a normal (left) and chronically disused (right) human vastus lateralis (VL) reflecting disuse-induced sarcolemmal disintegration in long-term bed rest (60 days BBR-2 study, Charité Berlin, 2007)
In skeletal muscle atrophy, a fiber-type specificity exists for the regulation of muscle mass. For example, fast glycolytic fibers (type II) are more vulnerable than slow oxidative fibers (type I) that correlated with distinct signaling pathways, for example, signaling transduction of forkhead box-O family transcription factors (FOXO), autophagy inhibition, transforming growth factor-beta (TGF-ß family), and nuclear factor-kappa-ß (NF-kB) expression (Wang and Pessin 2013). The resistance of oxidative fibers to atrophy may be explained by the protective mechanisms elicited by peroxisome proliferator-activated receptor gamma coactivator 1-alpha, PGC1α (Olesen et al. 2010; Wang and Pessin 2013). A number of current reviews are available on the mechanisms modulating the muscle phenotype based on slow versus fast myofiber fiber patterns (Blaauw et al. 2013), on the molecular basis of muscle atrophy including the IGF/Akt/mTOR pathway and the myostatin/Smad pathways, on the non-lysosomal ubiquitin proteasome pathway of muscle protein degradation via the E3 ligase proteins MuRF1/Mafbx/atrogenin-1 (Jackman and Kandarian 2004; Murton et al. 2008; Bodine-Fowler et al. 1995; Bodine and Baar 2012), on the countermeasure impact on signaling pathways in disuse (Chopard et al. 2009), and on nutritional aspects and the still controversially discussed muscle protein accretion/wasting mechanisms (e.g., protein synthesis vs. breakdown) in disuse atrophy and the impact of nutrient supplementation against imbalanced muscle protein turnover in disuse (Stein and Blanc 2011) (Fig. 2.7).
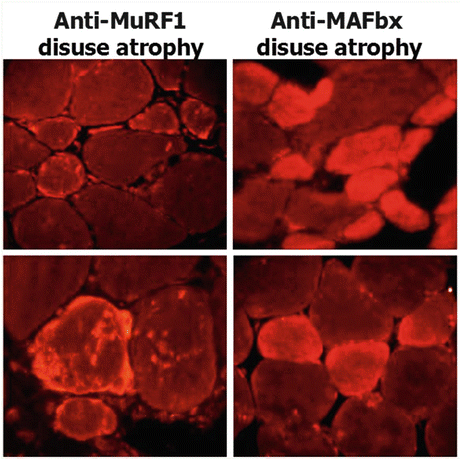
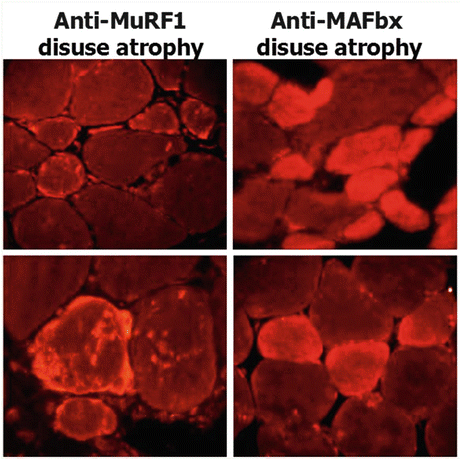
Fig. 2.7
MuRF1/MAFbx immunostaining (red) in cross-sectioned myofibers of disused normal human skeletal muscle fibers in female bed rest (2003 WISE-Study, Toulouse, France). Both immunolabels were used to visualize protein degradation (proteolysis) at the myofiber level following disuse (Courtesy: B. Schoser, LMU Munich (Salanova et al. 2008))
Skeletal muscle disuse normally shows a slow-to-fast fiber shift likely controlled by PGC1α expression and related signal pathways upregulated by exercise (Olesen et al. 2010; Handschin 2010; Peterson et al. 2011) likely affected by physical exercise as countermeasure (Desplanches 1997). An increased amount of hybrid fibers (co-expressing the slow and fast fiber phenotype, in the range of 5 < 15 %) compared to normal (2 < 5 % baseline) found in various atrophy models by slow/fast myosin heavy chain (MyHC) immunohistochemistry, immunoblotting, and proteomic analysis are considered as signs of fiber-type-related transient shifting and remodeling mechanisms in various muscle disuse paradigms without and with exercise as countermeasure (Salanova et al. 2008; Salanova et al. 2009b; Moriggi et al. 2010; Luxa et al. 2013; Sun et al. 2013).
The loss in muscle mass and the basic structural and functional consequences of unloading/disuse atrophy for skeletal muscle are well described (LeBlanc et al. 1992; Fitts et al. 2001). For example, in atrophic fibers following disuse/unloading, the fiber cross-sectional area (FCSA) and the myonuclear numbers are diminished (Ohira et al. 2002), and the fiber-type distribution in a given unloaded muscle shifts from slow to fast (fiber shift or transition) resulting in reduced force-generating capacity. In gravitational unloading in animals (HU-rats), the percentage of fibers expressing fast MyHC isoforms increases in unloaded soleus but not in fast muscles suggesting that predominantly slow muscles are more responsive to gravitational unloading than predominantly fast muscles (Ohira et al. 2002).
Even if extremely useful as a ground-based spaceflight analogue (Booth 1994), disuse in bed rest may be an extreme model of physical inactivity (Thyfault and Booth 2011), for example, to investigate the changes following sedentary lifestyles or even aging of modern societies. However, bed rest immobilization appears to also mimic many of the structural and functional changes observed in sedentary lifestyle residents (loss in muscle mass and force, others) either challenged by extended sitting time or much to low daily stepping patterns (<3,000 steps/day/healthy adults) compared to a physically active lifestyle with approximately > 7.000 steps/day of a recommended physical activity (Tudor-Locke et al. 2011).
However, the actual fiber composition in a normal healthy skeletal muscle largely depends on genetic predispositions and may vary due to lifestyle, history of activity, and sex and age of a subject (Baldwin and Haddad 2002). After analyzing more than 800 muscle biopsies from mainly slow and slow-/fast-mixed muscle types of healthy human subjects over the last decade, we suggest that apart from the known intersubject variability and sex differences, for example, between male and female muscle, a continuum of the myofiber-type distribution should be considered because of ongoing adaptation processes throughout normal human life on Earth. In laboratory rats and mice of comparable genetic strains, the muscle fiber size and type changes are “more stable” due to controlled housing conditions in standard vivarium cages (IVC cage) with defined group sizes, activity status, and feeding of standardized nutrients. Even smaller cages (<50 % reduced floor area vs. IVC cages) may not confound structural biochemical and behavioral analysis of laboratory mice (C57Bl/6 strain) even under fully automated life support housing conditions usually required for rodent spaceflight experiments (Blottner et al. 2009).
Due to the relatively well-known fiber-type composition of human skeletal muscle and due to their well-palpable anatomical location in the human body, two of them, the vastus lateralis and the soleus, have been considered by many laboratories around the world as standard reference muscles for the reliable statistical analysis in previous bed rest studies and most probably also in crew members for comparability reasons of ground and flight sample analysis.
2.1.7 NO Signals in Muscle: Nitric Oxide (NO) Produced by NO-Synthase (NOS) as Multifunctional Signals in Normal Muscle Physiology
With the discovery of nitric oxide (NO), formerly known as endothelial-derived relaxation factor (EDRF), biological gas signals were of particular interest in fundamental muscle biology and physiology (Bredt and Snyder 1990), disease (Brenman et al. 1995), clinical applications (Zhou and Zhu 2009), and, more recently, also in sports and exercise biology (Suhr et al. 2013). Biological NO signals were described in the late 1980s (around 1987–1989) as a completely new class of diffusible gaseous signals first described in vascular endothelial cells (NO as vasodilator) and in subsets of neuronal cells (NO as diffusible neurotransmitter, long-term potentiation) of the brain by several scientists including Salvador Moncada from the UK, but also by the US scientists Louis Ignarro, Ferid Murad, and Robert Furchtgott (Nobel Prize in physiology and medicine in 1998). In addition to NO signals found in the vasculature (endothelial NOS, eNOS) and central and peripheral neuronal networks (neuronal NOS, nNOS), skeletal muscle soon was identified as another large source of NO signals in the body (Nakane et al. 1993; Silvagno et al. 1996) including ventilatory muscles (Hussain et al. 1997). The skeletal muscle-derived NO signals and their dynamic roles in contraction and force mechanisms, myocellular metabolism, and cell signaling pathways became of particular interest for skeletal muscle biology and various adaptation mechanisms related to muscle structure and physiology. Currently, at least three individual NO-synthase genes are known, termed NOS1 (nNOS, neuronal), NOS2 (iNOS, inducible), and NOS3 (eNOS, endothelial) according to the proposed new terminology for NOS isoforms (Stamler and Meissner 2001) with about 20 different splice variants (Brenman et al. 1997) (Fig. 2.8). A muscle-specific splice variant of NOS1, termed mμNOS (Silvagno et al. 1996), was characterized as part of the dystrophin-glycoprotein complex of the skeletal muscle fiber membrane (sarcolemma) where mμNOS/NOS1 is anchored through a PDZ motif at its NH2 terminus to alpha and beta syntrophins and thus to dystrophin and dystrobrevin (Grozdanovic and Baumgarten 1999; Percival et al. 2008).
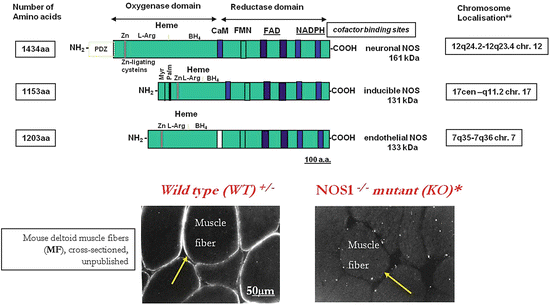
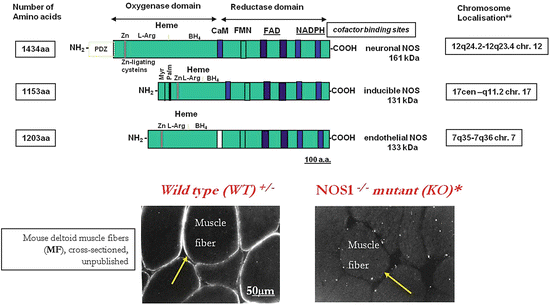
Fig. 2.8
Domain structure of NOS 1–3 genes on three human chromosomes. Skeletal muscle sarcolemmal nNOS immunolocalization (arrows) in wild-type versus nNOS−/− mutant mice deltoid muscle fibers (cross sections), D. Blottner, unpublished. Cartoon (upper panel) mod. from Ignarro LJ (ed) Nitric oxide biology and pathobiology, Academic Press, p.12 (2000)
NO signals are considered as metabolic regulators during muscle exercise in health and disease (Kingwell 2000) but also during muscle denervation and reinnervation (Tews et al. 1997; Rubinstein et al. 1998) and in muscle repair and remodeling mechanisms via satellite cells (Anderson 2000). Imbalance in NO signal mechanisms results in movement impairments such as slowing the walking speed in rats following in vivo NOS inhibition (Wang et al. 2001). Our previous basic research on the muscular NOS/NO system aimed at investigating the spatiotemporal expression and localization patterns using immunofluorescence methods with isoform-specific NOS antibodies in conjunction with nonradioactive NOS mRNA in situ hybridization techniques in skeletal muscle development that showed that all three major NOS1-3 isoforms are basically expressed in mouse C2C12 single myoblasts that are transiently upregulated when the single-nucleated myoblast fusion to become elongated multinucleated myotubes in vitro that eventually differentiate into mature skeletal muscle fibers (Blottner et al. 1998). Other work showed that explanted single muscle fibers are able to release measureable NO signals into the culture medium upon in vitro drug administration (NO-donors, arginine, or nitroprusside, Balon and Nadler 1994) and following in vivo electrical stimulation in the rabbit possibly involved in metabolic control of fiber phenotype transformation of anterior tibialis and extensor digitorum muscle (Reiser et al. 1997). In addition, mechanical loading was found to affect NOS expression by in vitro (myotubes) and in vivo (HU-rat) experiments confirming activity-dependent muscular NO signaling (Tidball et al. 1998; Fujii et al. 1998). Other studies in adult animals found that the sarcolemmal NOS is able to translocate from membrane to the sarcosol (cytosol) in response to mechanical unloading (Suzuki et al. 2007). Interestingly, the NOS2 isoform (inducible NOS) was found to be associated with the outer membrane protein caveolin-3 in slow-twitch guinea pig muscle suggesting involvement, for example, in glucose metabolism (Gath et al. 1999), a finding that was confirmed by our group in normal human muscle by altered NOS2-caveolin-3 co-expression following bed rest (Rudnick et al. 2004). In cultured C2 myotubes (mouse C2 cell line), we showed that NOS1 proteins co-cluster together with nicotinic acetylcholine receptors (major component of cholinergic synapses) by agrin-induced postsynaptic membrane clusters in vitro (Lück et al. 2000; Blottner and Lück 2001), and upon motor nerve contacting, the myocellular NO signals were upregulated in C2 myotubes in nerve-muscle cocultures performed in our laboratory (Püttmann et al. 2005). Nitric oxide synthase is expressed at the adult neuromuscular synapse (Kusner and Kaminski 1996) during development suggesting multiple signaling functions for neural transmission from motor nerve to skeletal muscle fiber (Blottner and Lück 2001). These studies showed that muscular NO signals are apparently regulated by neuronal mechanisms and should therefore have crucial signaling functions in postsynaptic neuromuscular control of skeletal muscle (Oliver et al. 1996).
Earlier work from our laboratory also suggested a sarcolemmal NOS1 localization as shown by NOS immunohistochemistry and also by the NADPH diaphorase enzyme histochemical activity (histochemical marker of NOS activity) in developing rat and mouse normal skeletal muscle by our group (Blottner and Lück 1998; Lück et al. 1998). In those studies, we found a gradient of increased sarcolemmal NOS expression in developing mice muscle fibers before and around birth (perinatally). In further animal and human studies, investigations on the muscular NOS/NO system turned out to serve as a valuable biomarker to study disuse atrophy (loss and/or translocation of myocellular NOS) and to assess efficacy of exercise countermeasure (preservation of myocellular NOS) in bed rest (see Sect. 2.2.3.4).
2.2 Lessons Learned from Ground-Based and Spaceflight Experiments
2.2.1 Animal Studies on Earth
Up to now, established and current in vitro models use isolated cells and tissues and multipotent stem cells cultivated in defined nutrient solutions under controlled conditions in special laboratory incubators. They are useful tools to study fundamental mechanisms in cell biology and physiology including cell development, differentiation, and growth, for example, triggered by bioactive molecules (growth factors, cytokines) by pharmacological in vitro cell assays as standard methods routinely used in research laboratories around the world. In gravitational and radiation biology, isolated primary cells, cell lines, or tissues are used to study microgravity changes in plant and animal cell biology grown in special cell incubators as payload to study fundamental mechanisms “unmasked of gravity” (Unsworth and Lelkes 1998), for example, cell shape and cytoskeleton changes in gravisensing (Hughes-Fulford 2003), bone cell signaling (Hughes-Fulford 2004), and cartilage differentiation (Duke and Montufar-Solis 1999), just to mention some of the cell biology experiments already flown in Space.
Skeletal muscle research offers a battery of myogenic cells (myoblasts, myotubes), isolated muscle fibers, and muscular stem cells (satellite cells) that are currently available to study defined aspects of physiological and pathophysiological mechanisms (e.g., fiber contractility, muscle development, and regeneration on Earth (Aas et al. 2013) as well as in simulated microgravity (Benavides Damm et al. 2013) and in spaceflight experiments (Franzoso et al. 2009).
However, isolated single cells or cell layers from various tissues sources (bone, muscle, brain, others) may not fully replace in vivo studies in small laboratory animals (rats and mice) as, for example, currently demonstrated by a successful stem cell therapy (stem cells were cultivated in vitro and afterward implanted in vivo) to offset skeletal muscle atrophy in mice (Artioli et al. 2014).
In vivo studies are essential to further understand the complexity of integrative signaling processes and their adaptation mechanisms inherent to the neuromuscular system in a normal living higher vertebrate organism including also humans. Due to cost limitations and other spaceflight constraints (design of animal housing devices for spaceflight), most of the animal studies in space research are performed by international scientific laboratories on the ground with expert staff and adequate equipment in animal facilities according to commonly accepted regulations for use and care of animals in scientific research consented and approved by national and local ethical board members of various disciplines (NRC 1996; Katahira 2001).
2.2.1.1 Ground-Based Experiments I: Hind Limb Unloading (HU) in Rats
Hind-limb unloading (HU) of the rat is a traditional and well-established ground-based experimental animal research paradigm in spaceflight research used by NASA to study disuse atrophy in small rodents such as rats (Morey-Holton and Globus 2002) or mice (Ferreira et al. 2011). Experiments with the HU-rat model require specially designed cages as animals need to move freely with both fore paws touching the ground for water and food ad libitum while the rat’s body is in a 30° angle to secure for hind-limb unloading (HU position, i.e., tail suspension) with both hind limbs freely hanging down without touching the bottom of the cage. Due to the common regulations of animal use and care in research, only laboratories with specialized animal facilities are able to use rats or mice over several days or weeks for HU experiments (Morey-Holton and Globus 2002). Because of ethical reasons, HU animal studies should be performed in cooperation between laboratories for optimal tissue sharing and analysis (Morey-Holton et al. 2007).
In a bilateral and interdisciplinary university cooperative between the Charité Berlin, Germany, and the Beihang University Beijing, China, we were able to share muscle tissue from a rat 21d HU experiment performed with a custom-made stepper device for rodents by our Chinese partners from the Key Laboratory of Biomechanics and Mechanobiology at the Beihang University to study bone and muscle loss under active versus passive muscle training (Sun et al. 2013). We found that the NOS1 biomarker in HU-rat soleus muscle almost disappeared from the unloaded myofiber sarcolemma (after 21 days of HU) compared to control animals (vivarium control). This loss in sarcolemmal NOS 1 was, however, prevented by active stepper training of hind limbs during HU position (reflexive muscle contractions triggered by plantar electric pulse arc stimulation) and to lesser extent also by passive stepper training during HU (passive mode motions induced by up and down movements of hind legs during HU via motorized foot pedals) compared to relevant controls performing comparable stepper training in normal body position in vivarium standard cages (Sun et al. 2013). This direct comparison between active and passive motions of disused rat skeletal muscle can be experimentally investigated only by the HU protocol that provided conclusive evidence for the presence of an activity-driven sarcolemmal NOS1 translocation from the myofiber sarcolemma to the sarcosol that can be offset by adequate muscle training (Sun et al. 2013). The results are interesting because activity-driven NO signals control a set of functional muscle-specific proteins via protein-S-nitrosylation, and, for example, abundant NO signals (too much or too little) could explain some of the altered muscular functions observed in human skeletal muscle disuse by nitrosative stress mechanisms experimentally proven in bed rest by our laboratory (see Sect. 2.2.3.5). In the same HU experiment, active stepping of gravitationally unloaded rat hind limbs prevented loss in tibial bone mass (the soleus originates from to the tibia) and trabecular microarchitecture, supporting the notion for optimization of human countermeasure protocols that should be targeted to individual human muscle-bone units following disuse on Earth, in rehabilitation, or in spaceflight.
2.2.1.2 Ground-Based Experiments II: Vestibular Deafferentation in Rats
As already discussed in the introduction chapter, the vestibular system (organ of equilibrium) is a key equilibrium and spatial reference system for adequate body movement control on Earth that undergoes considerable modifications during spaceflight with obvious consequences on spatial orientation and well-being in crew members who, for example, may suffer from space motion sickness (Lackner and Dizio 2006). Previous findings from the literature suggested that the vestibular outflow may control general sympathetic outflow in the body and, in particular, also the muscular sympathetic nerve activity on Earth as well as in Space. For example, baseline sympathetic outflow was found to be increased as a reason for hemodynamic stress in humans in Space (Eckberg et al. 2003); human sympathetic nerve activity is reduced after short microgravity exposition (parabolic flight) and upregulated after longer periods of microgravity (spaceflight) with impaired arterial baroreflex (Mano and Iwase 2003; Mano 2005) concomitant to altered human muscle sympathetic nerve activity (Ertl et al. 2002). However, the obvious role of vestibular changes for skeletal muscle disuse atrophy received only little attention (Kasri et al. 2004; Tothova et al. 2006).
In a laboratory cooperation between the Physiology Department at the University of Caen, France, and the Neuromuscular Group at the Charité Berlin, we tested the hypothesis that the vestibular system may affect both myofiber size and type composition of skeletal muscle in normal adult rats (n = 8) subjected to vestibular deafferentation (labyrinthectomy) in a 30-day long laboratory experiment established by our French partner laboratory. This experiment made use of surgical removal of the vestibular system in rats or mice to extinct the gravity stimulus by destruction of the gravisensor on Earth (Jamon 2014). Compared to sham-treated controls (with still intact gravisensors), in vivo bilaterally vestibular lesioned rats now missing the graviceptors showed significant myofiber size reductions in tissue samples of postural soleus muscle, altered fiber-type composition, and reduced myonuclear NFATc1 transcription factor accumulation (i.e., NFATc1 shift from cytosol to nucleus), all signs of slow myofiber atrophy remodeling and transcriptional activity changes in slow myofibers of postural soleus muscle (Luxa et al. 2013). Interestingly, vestibular lesions also resulted in considerable bone loss in this animal model (Denise et al. 2009) with clinical implications considering the identification of a unique inner ear signal control of bone formation (Vignaux et al. 2013) and possibly also acting on skeletal muscle quality (Luxa et al. 2013). Even if the exact neuroanatomical links remain to be elucidated, it seems likely that, similar to bone formation, skeletal muscle adaptation may be controlled by autonomic nervous system signals via spinal vestibulosympathetic outflow to peripheral targets to support normal skeletal muscle mass and function under terrestrial gravity (Luxa et al. 2013). These findings are of particular interest to find more adequate countermeasures against microgravity-induced muscle atrophy and bone loss for the crew members in future spaceflights. These findings also may have some impact on the clinical management of common vestibulosympathetic disorders (Ménière’s disease), for example, for better interpretation of clinically feasible skeletal muscle monitoring by vestibular evoked myogenic potentials to reliably determine inner ear vestibular saccule function (Honaker and Samy 2007).
2.2.2 Animal Studies in Spaceflight
Since the early days of spaceflight, animals were frequently flown in Space by NASA and the Russian Space Agencies onboard satellites on orbit (Cosmos) for several days up to a few weeks (Morey-Holton et al. 2007; Ballard and Rossberg Walker 1992; Oganov et al. 2006). A review on the history of spaceflown animals “from the first dog to the last monkey in space” can be found elsewhere (Ilyin 2007). Animal experiments in space with higher vertebrates and mammals are essential to study the physiological mechanisms working in the human body in microgravity (Yamasaki and Shimizu 2004) in sufficient statistical numbers. Our group participated to an international muscle team involved in two flight experiments with rodents in Space, one in 2009 (MDS mission, Sect. 2.2.2.1) with mice housed for over 90 days in a special animal payload on the ISS (mice drawer system, MDS, 2009), supported by the Italian Space Agency (ASI), NASA, and JAXA, and another one in 2013 with adult mice flown on a Russian biosatellite (BION-M1, Sect. 2.2.2.2), supported by the DLR (Germany) and IMBP (Russia).
It should be noted that mice are housed in Space in smaller cages (reduced area cages) than normally used in animal vivarium on the ground (standard ICV cages) with the potential risk of confounding results obtained by spaceflight experiments with mice living on reduced area (floor size) with, for example, less activity levels and challenged by higher stress levels (noninvasively analyzed by corticosteroid monitoring of feces). However, pilot laboratory experiments with C57/Bl6 mice housed in automated life support cages with reduced floor areas (MRSM cages, Fig. 2.9) for 3 weeks at the laboratory of our cooperation partners of the Measure, Model, and Manage Bio-Responses (M3-BIORES) working group at the University of Leuven (Kastelpark Arenberg, Heverlee), Belgium, revealed little if any negative effects on musculoskeletal system and behavioral outcome (stress and learning) produced by the unique cage design (Fig. 2.9) suggesting that the new environmental condition may not confound structure, physiology, and behavior results from Space Life Sciences research experiments and suggesting that mice preadapted to fully automated life support animal habitats for about 1 week prior to launch (astromice) should be implemented in future scenarios of spaceflight experiments (Blottner et al. 2009).
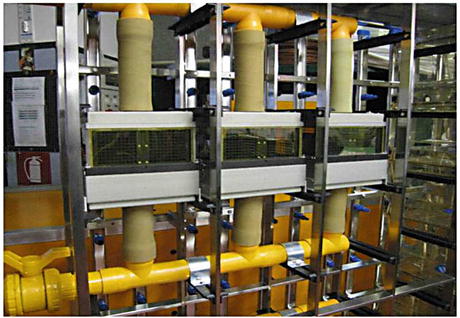
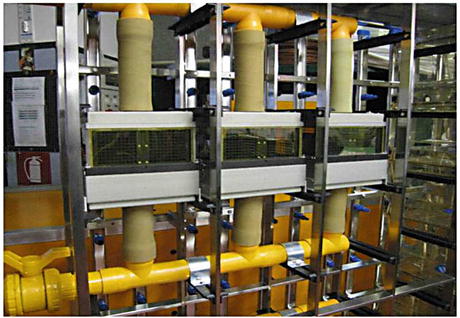
Fig. 2.9
Ground-based mouse science reference module (MRSM) experiment performed together with an ESA/ASI supported multidisciplinary MISS Facility Science Team (FST) of scientific experts (L. Vico, M. Jamon, D. Berckmans, D. Blottner), ESA representatives (P. Schiller, O. Angerer, J. Hatton, F. Gaubert), ASI representatives (V. Cotronei), and industrial partners from Thales-Alenia Space (G. Falcetti) located at the Catholic University of Leuven, Belgium, in 2006–2007. Biological testing was performed for any structural, physiological, and behavioral parameter changes of male C57Bl/6 mice housed (singly or in pairs) in small animal habitats (cf. three reduced floor size cages seen in middle racks) compared to standard-sized IVC cages integrated in the same rack shown (at the right side). Each mice habitat (cage) has an automated food supply system on its rear wall, a waste filter system underneath the cage bottom. Cages are air-conditioned through a closed tube system connected to an automated life support system (not shown) for ambient air ventilation, temperature, and humidity control (Blottner et al. 2009)
2.2.2.1 Mice on the International Space Station (ISS). MDS Mission
In 2009, a spaceflight payload for rodent research on the ISS, the mice drawer system (MDS), was initiated by the Italian Space Agency (ASI) and designed by Thales Alenia Space Italia company (Fig. 2.10). The MDS payload was flown to the ISS via the Shuttle Discovery 17A/STS-128 (Aug 28th, 2009) and returned to Earth with Shuttle Atlantis ULF3/STS-129 (Nov 27th) after 91 days in Space, which currently still is the longest permanence of mice in Space ever (Cancedda et al. 2012). The MDS housed six mice (3 wild-type and 3 PTN-Tg mutant mice, overexpressing a bone-specific promoter for pleiotropin (Fig. 2.10). Unfortunately, three mice died during the MDS mission due to health status and payload-related reasons. The remaining mice showed a normal behavior during the experiment, food and water and health status were daily checked, and they appeared in excellent health after landing. To receive as much information as possible on the microgravity-induced changes, spaceflown mice were immediately sacrificed upon return from Space, the various tissues of interest dissected within 24 h after landing and distributed to a Tissue Sharing Team of about 20 research teams from 6 countries including Germany (Charité Berlin). A ground replica of the flight experiment including animal housing in a second ground-based MDS drawer device was performed in parallel to the spaceflight experiment in the PI’s laboratory (R. Cancedda) of the University of Genova, Italy. In addition, control tissue was also obtained from mice housed in standard vivarium cages on Earth (Cancedda et al. 2012).
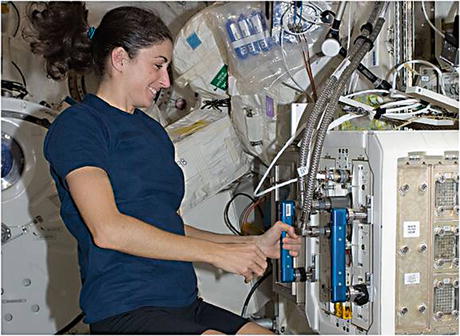
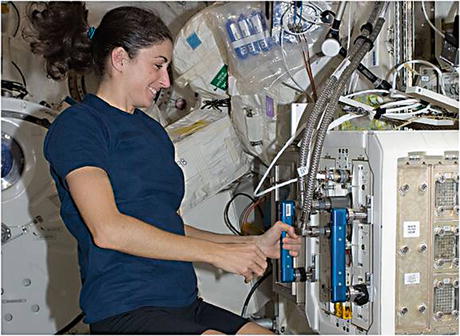
Fig. 2.10
The 91 days duration mice drawer system (MDS) module scenario (Aug 28 to Nov 27, 2009, STS 128/129, PI Cancedda) on the International Space Station (ISS). NASA Astronaut Nicole Scott onboard the ISS at the Japanese Kibo Laboratory with the MDS module (right side) housing three (n = 3) C57Bl/10 J and three (n = 3) osf-1 transgenic mice (Courtesy: NASA (http://onorbit.com/node/1601))
The skeletal muscle changes of the MDS mice include a slow-to-fast transition of the soleus myofibers (type 1/type 2 shift) and slow/fast myosin heavy chain content typically for this postural muscle following extended microgravity unloading. The sarcolemmal NOS1 translocation to the sarcosol was also found after prolonged microgravity exposure (Sandona et al. 2012) as well as flight-related NOS1–3 gene expression in a muscle-specific pattern (SOL vs. EDL) by microarray analysis (Fig. 2.11) confirming the previous ground-based experimental results reported by our group exposed to simulated microgravity models in animals (HU) and humans (bed rest). Moreover, a set of atrophy-related ubiquitin ligases (MuRF E3 ligase), sarcolemmal ion channels (NAV1.4, K + −channel subunits Kir6.2, SUR2A, and SUR1), various stress-related genes (NF-kB), and transcription factors (MRF-4) were upregulated in spaceflown soleus muscle compared to ground controls (Sandona et al. 2012). In conclusion, antigravity muscles such as the soleus react in a very sensitive way against prolonged microgravity exposure, while other calf muscles (EDL) were more resistant to unloading probably by activating compensatory and protective signaling pathways. These results support the idea to identify molecular targets for the new development of countermeasures (Sandona et al. 2012). Some of the results of the MDS mission, for example, on bone, muscle, and brain of the spaceflown mice including our data on skeletal muscle, are published elsewhere (Cancedda et al. 2012; Ohira et al. 2014; Santucci et al. 2012; Tavella et al. 2012; Sandona et al. 2012; Camerino et al. 2013).
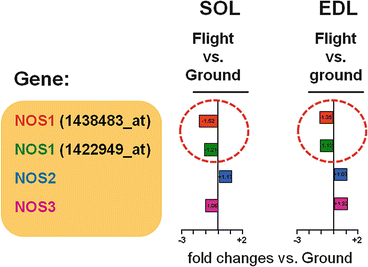
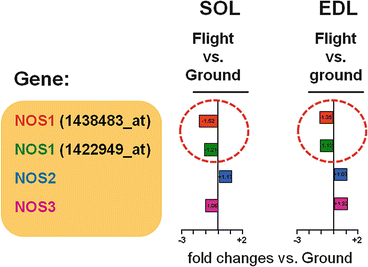
Fig. 2.11
Nitric oxide synthase isoform (NOS1-3) gene expression (microarrays) in mouse soleus (SOL) and extensor digitorum (EDL) skeletal muscle following long-term spaceflight (flight) onboard the ISS (91d MDS mission) compared to ground controls (ground) (Manuscript in preparation)
2.2.2.2 Mice Onboard a Biosatellite: 30 Days BION-M1 Flight Campaign
The BION satellite program for investigations of microgravity changes on various biological organisms and organ systems of higher vertebrates has a long tradition in Russian spaceflight research (Ilyin 2000). In a university cooperation between the Charité Berlin, Germany, and the Institute of Biomedical Problems (IMBP) of the Russian Academy of Sciences, Moscow, Russia (kindly supported by DLR/BMWi grants), our laboratory had the exciting possibility of participating to a 30-day BION-M1 flight campaign with young adult male mice housed separately by groups of six animals per cage in a controlled animal life support device with automated food and water supply on a biosatellite flown to orbit by a Soyuz-2 rocket (Andreev-Andrievskiy et al. 2014). The flown mice that returned back to Earth in life and in good health were sacrificed 24 h after landing by a dissection team at IMBP, and various tissues were distributed to each laboratory according to a special tissue preservation plan (sample preparation) and according to a dissection schedule consented by all investigators prior to end of the 2013 BION M1 mission (Fig. 2.12). Our laboratory received samples from six different skeletal muscles from five spaceflown mice each (n = 5, BION flight) and from a number of age- and sex-matched mice from three control groups (BION ground, flight control, vivarium control, each n = 6 or n = 8) that are currently being analyzed.
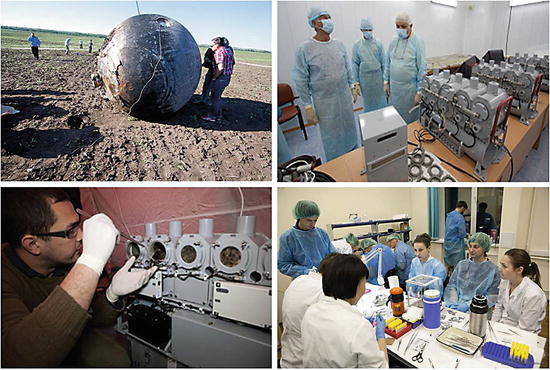
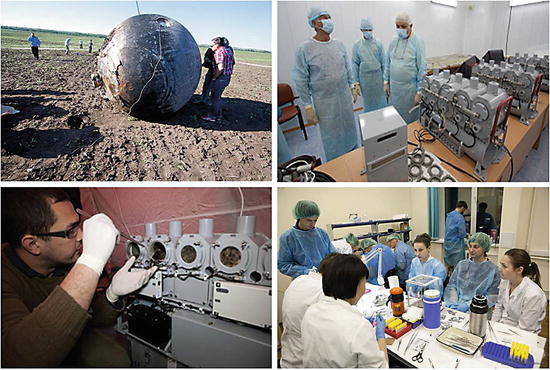
Fig. 2.12
The BION-M1 biosatellite flight experiment scenario (April 19 to May 19, 2013). The BION M1 biosatellite with mice and other biological samples was flown by SOYUZ-2 rocket to near orbit (575 km altitude, x477 orbital circulations) and returned to Earth (Kasachstan, Russia) after 30 days (upper left) with soft landing system. Flown mice were harvested on landing site and delivered to the IBMP, Moscow, 11 h post landing (upper right). Three C57Bl/6 SPF mice (aged 4–5 months, approx. 28 g b.wt. each) were housed in a small container (lower left showing 4 of 5 module containers) with food and water ad libitum, 12 h light/dark cycle, and under ambient environmental parameters (21 °C temperature, 60 % rel. humidity, pO2 140–180 mmHg concentration, pCO2 traces). Mice were sacrificed, and tissue samples prepared by a well-experienced team of scientists (lower right), frozen fixed, and delivered to the principal investigator’s laboratories (Images: IMBP, Moscow, Russian Federation)
Preliminary results obtained from first analysis of the BION samples confirmed that the postural soleus and the longissimus dorsi back muscle of flown mice are highly atrophic compared to ground-based controls (ms. in preparation). In addition, intensity of the NOS1 immunoreactivity is changed in the soleus muscle compared to ground controls confirming the presence of microgravity-induced control mechanisms related to the muscular NOS/NO system in mice previously found in HU-unloaded rats (Sun et al. 2013) but also in disuse atrophy in bed rest (Rudnick et al. 2004). Further analysis, for example, on the transcriptional level, gene expression, and various putative muscular signaling pathways involved in gravitational unloading mechanisms, is currently under way.
2.2.3 Human Studies (Bed Rest)
In bed rest studies, the human body is positioned recumbent in special clinic beds usually with six-degree head-down tilt, HDT (Fig. 2.13), to study the effects of immobilization on the unloaded healthy human body during or after short-term- (>7 days), medium-term- (>21 days), and long-term-duration (>60, 90, or 120 days) bed rest in controlled laboratory environments such as provided by transitional bed rest wards (infirmary rooms) provided by university hospitals (Charité Berlin) and more specialized bed rest facilities such as provided by the National Aerospace Institute, Cologne (DLR, :envihab, Germany), and the Medical Space Clinics (MEDES) in Toulouse, France, in Europe. The HDT supine position results in partly unloading of the body with a concomitant body fluid shifting from lower to upper body parts (centralization of venous blood). An increased preload (venous return flow to the right atrium of the heart) in the cardiovascular system results in endocrine stimulation (atrial natriuretic protein secreted from heart atrium) with an enhanced renal fluid and mineral (Na2+, Ca2+) excretion that is even more intensified by bone mineral turnover and loss during the bed rest period (LeBlanc et al. 2007).
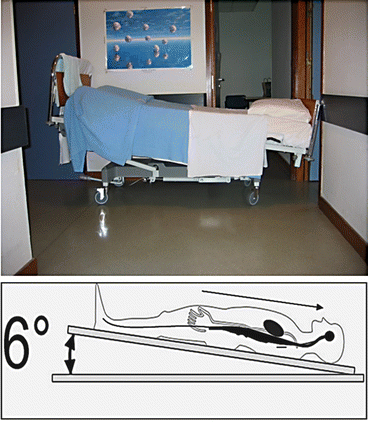
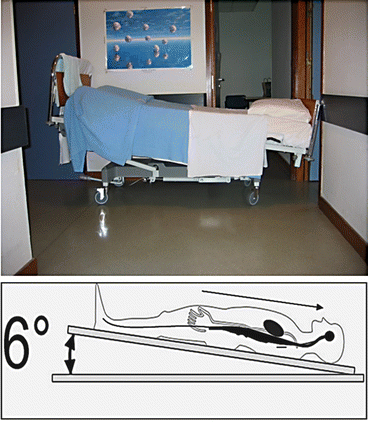
Fig. 2.13
A typical 6° head-down tilt (HDT) bed rest scenario/spaceflight analogue. Upper panel shows an empty bed at HDT position at the corridor of MEDES Space Clinics, Toulouse, France. Lower panel shows that volunteers at HDT supine body position are challenged by cephalad fluid shift (toward head) and partial unloading with disuse atrophy particularly of leg skeletal muscles throughout the entire bed rest period of weeks or months (spaceflight analogue model) (Images: D. Blottner)
Immobilization-induced human body unloading results in reduced muscle tone and neuromuscular activity (hypokinesia) of trunk, back, and leg postural (antigravity) muscles which also result in typical structural changes (e.g., muscle volume and myofiber size reduction, myofiber slow-to-fast-type transition, altered capillary-to-myofiber ratios, intermuscular adipose tissue) and functional changes (e.g., fatigue resistance, loss in power and force, reduced neuromuscular activity) observed after extended muscle disuse (Clark 2009). As a consequence, body immobilization with hypokinesia in HDT results in disuse-induced loss of bone and muscle mass (estimated atrophy rate 1–3 % per week HDT) with reduced muscle strength (power and force) and, finally, also impaired performance control similar to the known challenges observed in crew members in microgravity in Space or after return to Earth. Since decades, HDT bed rest has been considered as an experimental analogue to spaceflight on the ground that is controlled more easily and with statistically sufficient high numbers of voluntary subjects at more economical costs and with less health risks than for human experiments with crew members under real microgravity environments (Convertino et al. 1989; LeBlanc et al. 1997). At end of a bed rest study, the volunteers are slowly accustomed to an upright body position with the help of a tilt table (orthostatic stimulation) and become instantly part of a post-recovery and rehabilitation protocol that usually is completed after 6 months or 1 year following bed rest and that again may allow for recovery studies after reloading of the human body comparable to gravitational reloading studies in spaceflown crew members after their return to Earth. Even though musculoskeletal and neuromuscular changes found in HDT are largely the same found in spaceflight or after return thereafter, it is quite reasonable that a number of other microgravity challenges for the deconditioned human body in real space missions (e.g., radiation, stress, proprioception, autonomic dysregulation, immunosuppression, vestibular control) or, for example, extreme physical and/or cognitive inflight challenges of crew members (mission duties, extravehicular activities) may not be adequately simulated by the bed rest paradigm with “terrestrial astronauts” at 1 G, for example, simulating μG-induced changes in postural reflexes, sensorimotor behavior, and visual-vestibular neural stimulation (Reschke et al 2009). As animal study results may not be transferred 1:1 to human physiology, the use of countermeasures of various modes (e.g., exercise, nutrition) can be tested only in bed rest for their feasibility and effects on disuse-induced muscle and bone loss on Earth with the possibility for their implementation to future inflight countermeasure protocols during long-term space missions to the Moon or Mars (Pavy-Le Traon et al. 2006).
2.2.3.1 Methods I: Muscle Biopsy
Many of the structural changes and in particular the muscle-specific cell signaling adaptation in disused human skeletal muscle cannot be studied in body fluids (saliva, blood, urine) from subjects. In contrast to functional bone markers detectable in body fluids, reliable serological muscle loss markers are presently not available (Nedergaard et al. 2013). Therefore, in order to study structural adaptations including muscle-specific signaling in disuse, a small amount of muscle tissue (biopsy) needs to be taken from a well-palpable skeletal muscle at a well-known anatomic site with minimal risks of neurovascular injury (blood vessels and nerves) and with minimal to moderate risks for discomforts (e.g., pinching pain, local hematoma, infection, scar formation). A muscle biopsy can be harvested by an experienced operator from well-palpable leg muscles of voluntary human subjects or crew members using the well-established needle biopsy technique (Bergstrom 1975). The so-called Bergström needle consists of a small hollow needle (12–15 gauge size) that is used to percutaneously harvest a small muscle tissue sample after local skin cleaning and anesthesia without or with suction modification (Tarnopolsky et al. 2011). The local anesthesia using lidocaine with epinephrine (usually injected to skin, subcutis, and fascia to help control incision-related bleeding) may, however, confound molecular analysis in needle biopsies that should be avoided by a more careful injection approach (Trappe et al. 2013). The Duchenne-Bergström percutaneous needle biopsy technique routinely gains a rice corn-sized tissue sample of about 100 mg > 150 mg of tissue (single pinch) routinely used in many previous human physiology studies, in bed rest, but also in crew members (Fig. 2.14). Currently, thinner needles are available recovering about 4 mg of tissue (single recovery) from the human vastus lateralis or from the latissimus dorsi back muscle that may open up new anatomical sites and functional muscle types to be analyzed, for example, by molecular tools where a few mg of sample amount is sufficient enough for molecular analysis (Paoli et al. 2010). Alternatively, a Rongeur surgical forceps developed to cut away bone and tough tissues has been used for a biopsy of soft muscle tissue (<150 mg) through an approx. 1 cm skin/fascia incision following local anesthesia in bed rest studies (Rittweger J, personal communication). In all cases, routine medical wound care (medical plaster) and, if necessary, anti-scar bandage with pressure dressing are applied to the small skin wound on the day of biopsy. The quality of the small tissue sample (fiber orientation, fascia and connective tissue impurities, blood coagulate) is instantly inspected under a binocular microscope, while the sample is cleaned of non-muscle tissue, cut to smaller aliquots as required (e.g., according to histology, biochemical and molecular tissue preparations), immediately frozen in liquid nitrogen (or treated for other preservation methods required), and usually stored at minus 80 °C freezer (or in 4 °C refrigerator) for further sample analysis in a laboratory on site or at home.
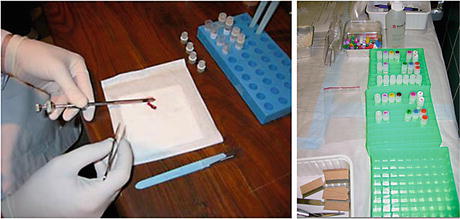
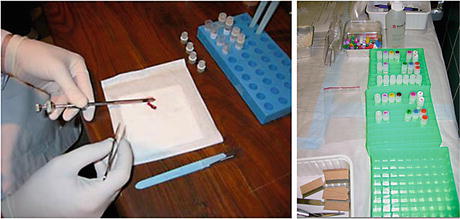
Fig. 2.14
Typical scenarios of human skeletal muscle biopsy tissue sampling (right, needle biopsy) and sample collection (left) using color-coded microcups for muscle team principal investigators (PIs). Image (left) taken from the 90 days LTBR study at MEDES, Toulouse, France, and (right) from the 60 days BBR-2 study at the Charité, Campus Benjamin Franklin, Berlin, Germany, 2007. In the BBR-2 study, an open incision biopsy procedure was used (Belavý et al. 2010) (Images: D. Blottner)
Due to sharp edges and scoop-shaped tips, both Bergström needle and Rongeur forceps share some risks of partial mechanical stress to the soft muscle sample that may interfere with a more broad tissue analysis including routine histology, ultrastructural analysis, and routine biochemical or sophisticated proteomic analysis. Some difficulties in biochemical and molecular measures caused by the needle biopsy protocol were reported that may result in variable changes in immunoblot signals, for example, when muscle samples were to be analyzed for cell signaling in human tissue (Caron et al. 2011). In order to minimize any mechanical stress during biopsy sampling and to improve tissue quality and quantity, an open incision biopsy technique has been alternatively used in two ESA long-term bed rest studies at the Charité Berlin (2003 Berlin Bed Rest (BBR)-1 and 2006–2007 BBR-2 study) that resulted in the sampling of muscle tissue of excellent quality (longitudinal fiber orientation) and quantity (>250 mg/biopsy) from the thigh (quadriceps femoris vastus lateralis) and the deep calf muscle (soleus). After local skin/fascia anesthesia, an approximately 1.5 cm skin/fascia incision is made, and a small bundle of muscle fibers is ligated and carefully separated from the adjacent fiber bundles of the muscle under visual control. Following proximal and distal cutting, the small ligated fiber bundle (approx. 300–400 fibers in longitudinal orientation) is then directly excised from the periphery of muscle by a surgeon, immediately handed over to another operator for further sample preparation, freezing, and storage. Meanwhile, both fascia and skin are sutured in situ (2–3 sutures) followed by normal wound care as shown in the BBR-2 study protocol paper (Belavý et al. 2010).
The open incision biopsy is a safe alternative to the standard needle biopsy but requires a well-trained surgeon and a surgery room of a clinic in a hospital. As already outlined, the latter technique has some advantages over the needle biopsy by getting “gold standard” biopsy samples from human skeletal muscle with much less mechanical manipulations and better histologic tissue preservation. High-quality biopsy material of sufficient amount is therefore proposed for comprehensive multidisciplinary human studies in future Space Life Sciences where ultrastructural/histological/high-resolution confocal laser structural analysis needs to be combined with molecular cell biology and sophisticated -omics technology (prote-/transcript-/metabol-/signal-omics) to get more comprehensive insights by using interdisciplinary tissue analysis to further understand the normal and abnormal mechanistic control and maintenance levels of disused skeletal muscle (Moriggi et al. 2010; Salanova et al. 2014) as a valuable on ground data base for comparison with the microgravity-induced changes in skeletal muscle atrophy.
The human skeletal muscle system is comprised of different muscle types with different functions (postural control/stabilization vs. fast mobilization) and different myofiber distribution patterns (type I, IIa, IIx) typical to a given muscle that appear to also respond in a typically muscle-specific way to disuse and exercise. Due to different physical training, routine daily activity patterns, endocrine status (sex hormones), and age, human skeletal muscle may show a high intersubject variability that should be considered for the study design in Human Space Life Sciences, for example, with study inclusion of sex- and age-matched subjects. For standardization, a biopsy is usually taken from at least two muscle types with a different fiber-type composition, e.g., the mixed fast/slow fiber composition thigh muscle (vastus lateralis) and the more slow fiber composition calf muscle (soleus), as reference muscles due to the fact that a wealth of structural and functional data was accumulated from these muscle types in many human studies on the ground and from spaceflight experiments over the last two or three decades.
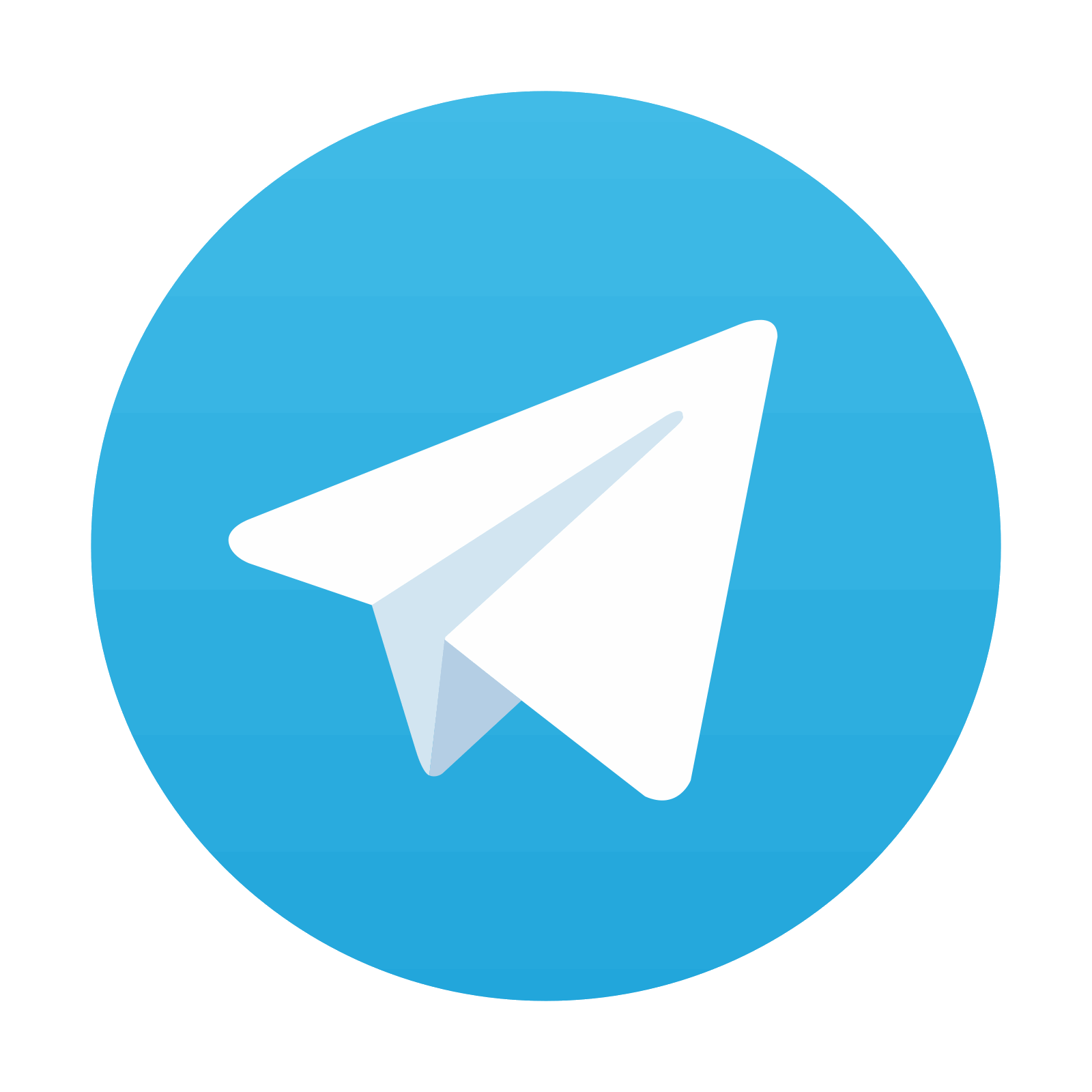
Stay updated, free articles. Join our Telegram channel
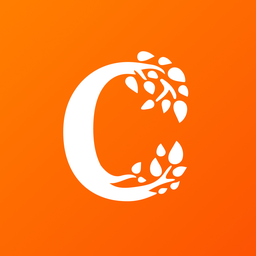
Full access? Get Clinical Tree
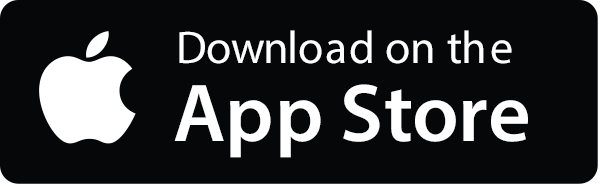
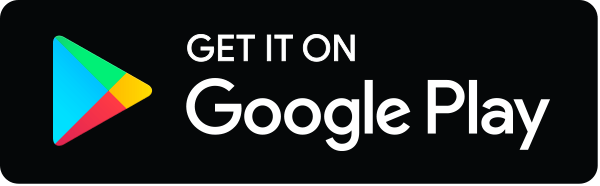
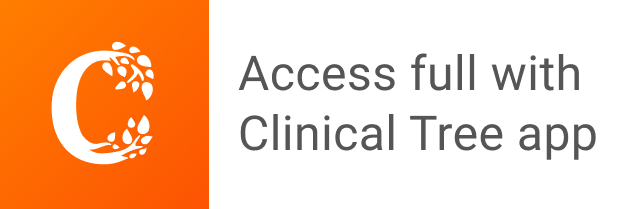