Fig. 41.1
Ascending Reticular Activity system (ARAS): The ascending arousal system. Two major pathways are shown. One (shown in yellow) providing upper brain stem input to the thalamic-relay nuclei and to the reticular nucleus of the thalamus coming from the pedunculopontine and laterodorsal tegmental (PPT/LDT) nuclei, which are acetylcholine (ACh)-producing neuronal groups. The second major group of neurons (shown in red) come from the noradrenergic (NA) locus coeruleus (LC), serotoninergic (5-HT) dorsal and median raphe nuclei, dopaminergic (DA) periaquiductal gray matter (vPAG), and histaminergic (His) tuberomamillary neurons (TMN). Additional cortical input merges from the basal forebrain (BF) neurons containing GABA or ACh, and by lateral hypothalamic (LH) peptidergic neurons that contain melanin-concentrating hormone (MCH) or orexin (hypocretin) (ORX). Reproduced from Saper et al. [29], with permission.

Fig. 41.2
Widespread projection of hypocretin
Neuroanatomic Substrates of REM Sleep
Transection experiments in cats through different regions of the midbrain, pons, and medulla [22, 30, 31] established the existence of REM sleep-generating neurons in the pons (Fig. 41.3). A transection at the junction of the pons and midbrain (level A) produced all the physiologic findings compatible with REM sleep caudal to this transection, whereas rostral to the section, in the forebrain region, the recording showed no signs of REM sleep. The structures rostral to a section between the pons and medulla (level B) showed signs of REM sleep but structures caudal to the section showed no signs of REM sleep. Following transection at the junction of the spinal cord and medulla (level C), REM sleep signs were noted in the rostral brain areas. Finally, transections at the pontomesencephalic (A) and pontomedullary (B) junctions produced an isolated pons that showed all the signs of REM sleep. The pons is, therefore, sufficient and necessary to generate all the signs of REM sleep.
To explain the mechanism of REM sleep, three animal models are available. The earliest is the McCarley–Hobson reciprocal interaction model (Fig. 41.4) based on reciprocal interaction of REM-on and REM-off neurons [1, 22] (see also Chap. 5). The cholinergic neurons in the PPT and LDT nuclei in the pontomesencephalic region are REM-on cells responsible for REM sleep, showing the highest firing rates at this stage. The aminergic neurons located in the LC and DRN are REM-off cells and are inactive during REM sleep. Histaminergic neurons in the tuberomammillary region of the posterior hypothalamus can also be considered as REM-off cells. Thus, the cholinergic REM-on and aminergic REM-off cells are all located within the transections of the pons as described previously. LDT-PPT cholinergic neurons promote REM sleep through pontine reticular formation (PRF) effector neurons, which in turn send feedback loops to the LDT-PPT. Cholinergic neurons of the PPT and LDT project into the thalamus and basal forebrain regions as well as to the PRF and are responsible for activation and generation of REM sleep. Aminergic cells play a permissive role in maintenance of the REM sleep state. In the latest modification of the reciprocal interaction model, McCarley [22] suggested that γ-aminobutyric acid (GABA) also plays a role in the REM sleep generation. At the onset of REM sleep, there is activation of GABA neurons in the pons that causes inhibition of the LC/DRN (REM-off neurons) as well as activation of (or disinhibition of) cholinergic neurons in the pons [32]. The reason for GABA activation is not known, and the source of GABAergic neurons is probably both local (e.g., a subgroup of PRF GABA neurons) and distant (e.g., GABAergic neurons in the ventrolateral periaqueductal gray). The theory for muscle hypotonia or atonia during REM sleep postulates that inhibitory postsynaptic potentials are generated by dorsal pontine interneurons in the region of the peri-LC alpha ventral to the LC that project to the lateral tegmentoreticular tract and then to the medial medullary region (the inhibitory zone of Magoun and Rhines in and around the nucleus magnocellularis and gigantocellularis in the paramedianus); the reticulospinal tract from this region projects to the anterior horn cells of the spinal cord, causing hyperpolarization and muscle atonia (Fig. 41.5) [1, 2, 33–37]. An experimental lesion in the peri-LC alpha region [38] as well as the medial medullary region [39] produced REM sleep without muscle atonia. In human REM sleep behavior disorder, causing dream-enacting behavior associated with REM sleep without muscle atonia, a structural or functional alteration of the pathway maintaining muscle atonia during REM sleep is most likely responsible [40].



Fig. 41.4
Schematic diagram of McCarley–Hobson model of REM sleep mechanism. GABA γ-aminobutyric acid; LC/DR locus coeruleus/dorsal raphe; LDT/PPT laterodorsal tegmental/pedunculopontine tegmental nuclei. Modified from McCarley [22]

Fig. 41.5
Schematic diagram to explain the mechanism of muscle atonia in REM sleep. GC gigantocellularis; LC locus coeruleus; MC magnocellularis; Peri-LC perilocus coeruleus alpha; RST reticulospinal tract; TRT tegmentoreticular tract
In the model, proposed by Lu et al. [41] (Fig. 41.6), there is a “flip-flop” switch interaction between GABAergic REM-off neurons in the deep mesencephalon, vlPAG, and lateral pontine tegmentum (LPT), and GABAergic REM-on neurons in the sublaterodorsal (SLD) nucleus, with a dorsal extension of the SLD named the precoeruleus. These mutually inhibitory neuronal populations (SLD GABAergic REM-on and GABAergic REM-off neurons in the deep mesencephalon–lateral pontine tegmentum) serve as a flip-flop switch. Ascending glutamatergic projections from precoeruleus neurons to the medial septum are responsible for the hippocampal electroencephalographic (EEG) theta rhythm during REM sleep. Descending glutamatergic projections from the ventral SLD directly to the spinal interneurons, apparently without a relay in the medial medulla, inhibit spinal ventral horn cells by both glycinergic and GABAergic mechanisms. Cholinergic and aminergic neurons play a modulatory role and are not part of the flip-flop switch. McCarley [22] suggested that this model is based on c-fos labeling only without electrophysiologic recordings. Furthermore, this model does not address how REM periodicity occurs in this flip-flop switch utilizing two mutually inhibitory neuronal populations. Finally, this model also does not explain the gradually increasing duration of REM sleep throughout the night and generally absent REM sleep during daytime naps.


Fig. 41.6
Lu-Saper “flip-flop” model shown schematically to explain REM sleep mechanism. eVLPO extended region of ventrolateral preoptic nucleus; GABA γ-aminobutyric acid; GLUT glutamatergic neurons; GLYC glycinergic neurons; LC + DRN locus coeruleus + dorsal raphe nuclei; LDT + PPT laterodorsal tegmental + pedunculopontine tegmental nuclei; LPT lateral pontine tegmentum; PC precoeruleus; SLD sublaterodorsal nucleus; VlPAG ventrolateral periaqueductal gray. Modified from Lu et al. [41]
In the model proposed by Luppi’s group [42–46] (Figs. 41.7 and 41.8), neurons active during REM sleep are identified in a small area in the dorsolateral pontine tegmentum called the sublaterodorsal (SLD) nucleus in rats (corresponding to the dorsal subcoeruleus nucleus in humans or peri-LC alpha region in cats). At the onset of REM sleep, there is an activation of REM-on glutamatergic neurons from the SLD. During NREM sleep and wakefulness, these neurons in the SLD would be inhibited (hyperpolarized) by tonic GABAergic input from GABAergic REM-off neurons located in the SLD, deep mesencephalic and pontine reticular nuclei, and ventrolateral periaqueductal gray (vlPAG) as well as monoaminergic REM-off neurons. Ascending dorsal SLD REM-on glutamatergic neurons can cause cortical activation through projections to thalamocortical neurons along with REM-on cholinergic and glutamatergic neurons from the LDT/PPT mesencephalic and pontine reticular nuclei and basal forebrain regions. Descending ventral REM-on glutamatergic SLD neurons would cause muscle atonia through excitatory direct and indirect projections to glycinergic and GABAergic premotor neurons in the magnocellularis and parvocellularis reticular nuclei in the medulla, causing hyperpolarization of the motor neurons. In the Luppi model, therefore, the glutamatergic neurons play a crucial role in REM generation. GABAergic neurons are responsible for inactivation of monoaminergic neurons during REM sleep, and cholinergic neurons do not play a crucial role in activating REM executive neurons in this model. In experimental REM deprivation studies in rats, Luppi’s group [42] showed that during REM recovery sleep, C-fos-activated SLD neurons were not cholinergic (i.e., there was no increase of choline acetyltransferase and the enzyme synthesizing acetylcholine) nor were they GABAergic (i.e., glutamate decarboxylase, the enzyme synthesizing GABA, did not increase in the majority of the SLD neurons). Most of the C-fos-labeled neurons localized in the SLD after REM recovery expressed vesicular glutamate transporter 2 (vGLUT 2), a specific marker of glutamatergic neurons. Therefore, SLD neurons triggering REM sleep are glutamatergic. The melanin-concentrating hormone (MCH) neurons in the lateral hypothalamus also participate in promoting REM sleep (see further on).



Fig. 41.7
Luppi Model. Schematic diagram of Boissard–Luppi model to explain REM sleep mechanism. DPGR dorsal paragigantocellular reticular nucleus; Dp-MES deep mesencephalic; GABA γ-aminobutyric acid; GLUT glutamatergic; GLYC glycinergic neurons; HYPO hypothalamus [hypocretinergic neurons in lateral hypothalamus]; LC-DRN locus coeruleus—dorsal raphe nuclei; MCRF magnocellular reticular formation; PCRF parvocellular reticular formation; SLD sublaterodorsal nucleus; Vl-PAG ventrolateral periaqueductal gray; VLPO ventrolateral preoptic region. Modified from Luppi and Fort [1] and [42]

Fig. 41.8
Neuronal network responsible for REM Sleep PS paradoxical (REM) sleep; BF basal forebrain; Ach acetylcholine; VLPO ventrolateral preoptic nucleus; GABA gamma amino-butyric acid; SCN suprachiasmatic nucleus; Hcrt hypocretinergic neurons; PH posterior hypothalamus; MCH melanin concentrating hormone-containing neurons; TMN tuberomamillary nucleus; HIS histamine; vlPAG: ventrolateral periaqueductal gray; dDPMe deep mesencephalic reticular nucleus; DRN dorsal raphe nucleus; 5HT 5-hydroxtryptamine; Ldt laterodorsal tegmental nucleus; PPT peduncutopontine tegmental nucleus; LC locus coeruleus; NA noradrenaline; SLD sublaterodorsal nucleus; Glu: glutamic acid; DPGi dorsal paragigantocellular reticular nucleus; Giv ventral gigantocellular nucleus; LPGi lateral paragigantocellular reticular nucleus; Rmg nucleus raphe magnus; Gia alpha gigantocellular reticular nucleus; Gly glycine. Reproduced with permission from reference [22] in the text
There are thus certain similarities and differences between these two contemporary models (Lu-Saper and Luppi) which can be summarized as follows: In both these models the pontine SLD neurons play a critical role. In the Luppi model the SLD REM-on neurons are glutamatergic, whereas in the Lu-Saper model GABAergic neurons in both REM-off and REM-on (SLD) regions play a crucial role in REM sleep by activating dorsal (for EEG) and ventral (for muscle atonia) glutamatergic pathways in the SLD. Both these models recognize the importance of glutamatergic system for generating EEG desynchronization and REM muscle atonia. In their initial report Luppi’s group suggested both a direct and an indirect projection from SLD to spinal cord via a relay in the VMM. The latest report [44, 45] from this group, however, suggested that REM muscle atonia is mediated mainly by a relay in the VMM and to a minor extent by a direct projection to spinal cord. Saper’s group, on the other hand, initially [41] suggested a direct projection from SLD to spinal cord without a relay in the VMM. In their latest [46] report this group modified their initial suggestion, now saying that REM atonia is mainly due to a direct spinal projection, but VMM may also play a minor role. The two models (Lu-Saper and Luppi) thus seem to be merging in finding a common ground to explain REM muscle atonia.
Luppi’s group [44] recently recognized the important role played by the lateral hypothalamus in REM sleep generation (see further on). They hypothesized that the melanin concentrating hormone (MCH)/GABAergic (REM-on) neurons in the lateral hypothalamus constitute a master generator of REM sleep by a direct inhibitory input to vlPAG region and deep mesencephalic (DPMES) reticular nucleus (REM-off GABAergic neurons) controlling onset and maintenance of REM sleep. Earlir Saper’s group [47] highlighted also the role played by the diffuse (extended) VLPO neurons of the hypothalamus in REM sleep generation by inhibiting vlPAG regions during REM sleep.
Brooks and Peever [48] initially challenged the glycinergic and GABAergic neurochemical mechanism of REM motor atonia based on microdialysis experimental evidence in rats that REM atonia persists even when glycine and GABA receptors are blocked and after simultaneous application of glutamatergic agonists to the trigeminal motor pool. These authors [49], however, reversed their conclusion and showed that addition of baclofen (a GABA b antagonist) to bicuculline (a GABA a antagonist) and strychnine (a glycine antagonist) during microdialysis restored muscle tone supporting the role of glycinergic and GABAergic neurons in REM motor atonia.
Neuroanatomic Substrates of NREM Sleep
Neurophysiologic studies of sleep really began after astute clinicopathologic observations by von Economo, who examined patients with encephalitis lethargica at the beginning of the twentieth century [50]. It was noted that lesions of encephalitis lethargica, which severely affected the posterior hypothalamic area, were associated with the clinical manifestation of extreme somnolence, whereas morphologic alterations in the anterior hypothalamic region were associated with sleeplessness. These observations led scientists to believe in the existence of the so-called sleep-wake centers [50–53].
Before the middle of the last century, the emphasis of sleep physiologists was on the passive [53–55] theories of sleep. Beginning in the late 1950s, thought shifted toward active sleep theories [3, 5, 56–65]. The passive theory postulates that sleep results from withdrawal of both specific and nonspecific afferent stimuli to the brain stem and the cerebral hemisphere. Proponents of active sleep theories suggest that activity of sleep-promoting neurons or the fibers of these so-called centers determine the onset of sleep. Most likely, proponents of both active and passive theories are partially correct, as far as the physiology and anatomy of sleep are concerned. These conclusions are based on stimulation, ablation, or lesion experiments. Later, these studies were extended to include extracellular and intracellular recordings and pharmacologic injections of chemicals into discrete areas to induce different states of sleep or to inhibit sleep [66].
The passive theory originated with two classic preparations in cats by Bremer [54, 67]: cerveau isolé and encephale isolé. Bremer found that midcollicular transection (cerveau isolé) produced somnolence in the acute stage and that transection at the C1 vertebral level, to disconnect the entire brain from the spinal cord (encephale isolé), caused EEG recordings to fluctuate between wakefulness and sleep. From these experiments, Bremer concluded that in cerveau isolé preparations, all the specific sensory afferent stimuli were withdrawn, and thus, sleep was facilitated, whereas such stimuli maintained the activation of the brain in encephale isolé preparations. These conclusions, however, have been modified since the discovery by Moruzzi and Magoun [55] in 1949 of the existence of nonspecific groups of neurons and fibers in the center of the brain stem called the reticular formation. Moruzzi and Magoun [55] stated that the brain stem ARAS energized the forebrain and that withdrawal of this influence in cerveau isolé preparation resulted in somnolence or coma. The observations of Moruzzi and Magoun [55] that EEG desynchronization results from activation of the midbrain reticular neurons, which directly excite the thalamocortical projections, have been confirmed by more recent intracellular studies [68, 69]. It was thought that wakefulness resulted from activation of the ARAS and diffuses thalamocortical projections [1]. After stimulation of these structures, the EEG shows diffuse desynchronization, whereas lesions in these structures produce EEG synchronization or the EEG NREM sleep pattern. This also supports the suggestion of Steriade et al. [70] that, at the onset of NREM sleep, there is deafferentation of the brain due to blockage of afferent information first at the thalamic level, causing the waking open brain to be converted into a closed brain resulting from thalamocortical inhibition. The reticular nucleus of the thalamus is responsible for the origin of the sleep spindles [1]. Stimulation of this nucleus produces spindle-like activity, whereas destruction of it abolishes the spindles unilaterally and bilateral destruction abolishes the spindles on both sides.
The passive sleep theories were challenged by findings that came in the wake of midpontine pretrigeminal brain stem transection in cats performed by Batini et al. [57, 58]. This preparation is only a few millimeters below the section that produces the cerveau isolé preparation. In contrast to the somnolence produced by the cerveau isolé preparation, the midpontine pretrigeminal section produced persistent EEG and behavioral signs of alertness. These observations imply that structures located in the brain stem regions between these two preparations (cerveau isolé and midpontine pretrigeminal) are responsible for wakefulness. Data demonstrate cholinergic neurons in the PPT nucleus and in the LDT nucleus in the region of the midbrain-pontine junction [1]. These groups of cholinergic neurons have been shown to have thalamic and basal forebrain projections as well as projections toward the medial PRF. These neurons are responsible for activation and for generation of REM sleep in the McCarley–Hobson model [1] (see Chap. 5). The forebrain cholinergic neurons from the basal nucleus of Meynert project to the cerebral hemisphere, particularly to the sensorimotor cortex, and lesions in these neurons disrupt the EEG waves and elicit diffuse slow waves [1]. The finding of cholinergic neurons at the mesopontine junction confirms the conclusions drawn by Batini et al. [57, 58] after midpontine pretrigeminal transections.
The active hypnogenic neurons for NREM sleep are thought to be located in two regions [1]: (1) the region of the nucleus tractus solitarius (NTS) in the medulla and (2) the preoptic area of the hypothalamus and the basal forebrain area (see Chaps. 5 and 8). Recently, another region in the medulla is thought to be promoting slow-wave sleep (SWS) active GABAergic neurons in the medullary parafacial zone (PZ) by releasing synaptic GABA onto parabrachial neurons which then excite basal forebrain by releasing glutamate and modulate cortical EEG [71]. The evidence is based on stimulation, lesion, ablation studies, extracellular and intercellular recordings [1], and expression of c-Fos protein immunoreactivity. The active inhibitory role of the lower brain stem hypnogenic neurons on the upper brain stem ARAS has been clearly demonstrated by Batini et al.’s [57, 58] experiment of midpontine pretrigeminal sectioning. Similarly, electrical [62] stimulation of the preoptic area, which produced EEG synchronization and a behavioral state of sleep, supported the idea of the existence of active hypnogenic neurons in the preoptic area [1]. Nauta’s [53] experiments in 1946 that showed insomnia after lesions of the preoptic region also supported the hypothesis of active hypnogenic neurons in the forebrain preoptic area. Experiments by McGinty and Sterman [64] in 1968 confirmed Nauta’s observations. More recently, ibotenic lesions in the preoptic region have been found to produce insomnia, and these results support the active hypnogenic role of the preoptic area [1, 72]. In the same experiments, however, injections of muscimol (a GABA agonist) in the posterior hypothalamus transiently recovered sleep, suggesting that the sleep-promoting role of the anterior hypothalamus is dependent on inhibition of posterior hypothalamic histaminergic awakening neurons. It is also notable that in 1934, Dikshit [73] induced sleep by intrahypothalamic injection of acetylcholine, suggesting the presence of a sleep center in the hypothalamus. Contemporary theory suggests that NREM sleep-promoting neurons are found in the VLPO area and the median preoptic nucleus (MnPn) [74] of the anterior hypothalamus as well as in the region of the NTS and the parafacial zone (PZ) in the medulla. VLPO neurons consist of two subgroups—“clustered” and “diffuse” or extended—depending on the distribution pattern [47, 75].
It has recently been shown that MCH neurons (GABAergic) also play an important role in promoting and stabilizing sleep along with VLPO and MnPn of the anterior hypothalamus [47, 74–78]. MCH neurons are intermixed and interconnected with hypocretin (orexin) neurons in lateral hypothalamus and receive inhibitory inputs from arousal neurotransmitter system (e.g., noradrenergic, serotonergic, histaminergic, and cholinergic) [78]. MCH neurons also project widely to other hypothalamic neurons as well as to the limbic system including the amygdala [79]. The hypothalamic preoptic (e.g., VLPO and MnPn) and MCH neurons are sleep-promoting neurons as evidenced by increasing firing of the units on intracellular recordings and increased expression of C-fos immunoreactivity in VLPO and MnPn as well as after optogenetic stimulation of MCH neurons [76, 78]. VLPO neurons are responsible for sleep consolidation and maintenance, whereas MnPn is responsible for sleep onset. Activation of VLPO and MnPn (using GABA and galanine inhibitory neurotransmitters) results in inhibition of the arousal systems in the brain stem (aminergic, serotonergic, and dopaminergic), forebrain (cholinergic), and posterolateral hypothalamus (hypocretinergic and histaminergic). Thus, there is a reciprocal interaction between sleep-promoting and wake-promoting neurons [74, 47]. The beginning and ending of the state may be determined partly by self-inhibition of the respective neurons and dissipation of sleep-promoting factors (e.g., adenosine, the cytokine interleukin-1β or IL-1β and prostaglandin D2 or PGD2). The strong anatomic connection between suprachiasmatic nucleus (SCN) controlling circadian regulation and VLPO-MnPn-MCH neurons responsible for homeostatic regulation suggests an important role for SCN in sleep-wake regulation, but their functional interaction is not clearly determined.
The tightly clustered VLPO neurons project to the tuberomammillary nuclei, inhibiting them and promoting NREM sleep, whereas diffusely distributed (extended) VLPO neurons along with MCH neurons project to and inhibit the aminergic nuclei in the LC and the dorsal raphe region of the brain stem as well as inhibiting the pontine GABAergic neurons in the ventrolateral periaqueductal gray (VLPaG)/SLD, participating in REM sleep [76, 77, 80]. It is notable that MCH neurons fire maximally during REM sleep and to a lesser extent during NREM sleep [76, 77]. In narcolepsy, loss of hypocretinergic neurons facilitates (disinhibits) MCH neurons, thereby promoting sleepiness and cataplexy.
The contemporary theory for the mechanism of NREM sleep thus suggests a reciprocal interaction between two antagonistic neuron types in the VLPO and MnPn of the anterior hypothalamus and MCH neurons in the lateral hypothalamus and wake-promoting neurons in the tuberomammillary nuclei of the posterior hypothalamus, as well as the LC, dorsal raphe, basal forebrain, and mesopontine tegmentum [1, 22, 47, 74, 75]. Reciprocal interaction between sleep-promoting neurons in the region of the NTS and wake-promoting neurons within the ARAS of the brain stem independent of the reciprocal interaction of the neurons of the forebrain also plays a role in the generation of NREM sleep, as stated earlier. In summary, the active and passive theories of sleep may be viewed as complementary rather than mutually exclusive mechanisms [1]. The role of postulated humoral sleep factors (e.g., prostaglandin D2, the cytokine interleukin-1β (IL-1β), growth hormone-releasing factor, and muramyl peptides) remains undetermined in the absence of experiments to test their role at the cellular level in critical brain areas. It has been suggested that adenosine, a neuromodulator, may act as a physiologic sleep factor modulating the somnogenic effects of prolonged wakefulness [81]. This has been postulated after experiments in cats have shown that adenosine extracellular concentration in the basal forebrain cholinergic region increased progressively during prolonged spontaneous wakefulness.
Many important unanswered questions remain regarding the mechanism of sleep. Why do VLPO and MnPn neurons fire at sleep onset? What initiates the cascade of disfacilitation in the brain stem wake-promoting neurons? What initiates activation of LDT-PPT neurons during REM? What causes activation of GABAergic pontine SLD neurons at the onset of REM sleep? What activates pontine glutamatergic neurons at REM onset? What causes activation of wake-promoting neurons at sleep offset? And, finally, what maintains NREM-REM cycling? We here provide a speculative summary to answer some of these questions. VLPO, MnPn, and MCH excitation at NREM sleep onset is initiated by progressive accumulation of adenosine (a sleep-promoting factor in the forebrain region accumulated during prolonged wakefulness) and probably also by an excitatory drive from the suprachiasmatic nucleus (SCN) as well as reciprocal inhibition of aminergic and orexin wake-promoting neurons; progressive inhibition of aminergic REM-off and hypocretinergic neurons causes disinhibition of REM-on neurons and initiates REM sleep; a simultaneous cascade of disfacilitation of the brain stem arousal system with decreased environmental afferent stimuli culminates in blockade at thalamic levels. Physiologically, facilitation (or disinhibition) after a certain period (perhaps determined in the case of sleep-wake regulation by the SCN regulatory neurons connected anatomically to sleep-wake neurons) will be followed by inhibition (or disfacilitation), i.e., these sleep-promoting neurons are self-inhibiting, and thus, the cycle will begin again. For additional discussion on the functional anatomy of sleep, the reader is referred to Chaps. 5 and 8.
Marked impairment of the arousal and cognition systems may result in coma or severe sleepiness. The reversibility of this state of awareness differentiates sleep from coma. There are also physiologic and metabolic differences between sleep and coma. Coma is a passive process (loss of function), whereas sleep is an active state resulting from physiologic interactions of various systems in the brain stem and cerebral cortex. Metabolic depression of the cerebral cortex and brain stem characterizes coma and stupor, whereas in sleep oxygen use and metabolic rhythm remain intact. By disrupting the arousal system and stimulating the sleep-promoting neurons, focal neurologic lesions may also cause excessive sleepiness. For example, lesions of the brain stem, thalamus, hypothalamus, and PAG regions may produce excessive sleepiness, stupor, and coma. These lesions may also affect lateral hypothalamic hypocretinergic neurons as well as REM-generating neurons in the pons and cause various REM sleep alterations including symptomatic narcolepsy.
Functional Anatomy of Respiration in Sleep and Wakefulness
The neuroanatomy of respiration, its control, and physiologic changes during sleep in healthy individuals are described in detail in Chap. 11. Briefly, respiration is controlled by the automatic or metabolic and behavioral systems [11–14, 82–86]. The two systems are complemented by a third system known as the arousal system, which may also be called the system for wakefulness stimulus [86, 87]. These respiratory systems work in concert with the various peripheral and central inputs to maintain acid-base regulation and respiratory homeostasis [9]. The location of the respiratory neurons makes them easily vulnerable to a variety of central and peripheral neurologic disorders, particularly central neurologic disorders involving the brain stem. Many acute and chronic neurologic illnesses may affect central or peripheral respiratory pathways, giving rise to acute respiratory failure in wakefulness and sleep. Some conditions may affect control of breathing only during sleep. Such a condition may cause undesirable, often catastrophic, results, including cardiorespiratory failure and even sudden death.
Sleep-Related Respiratory Dysrhythmia in Neurologic Disorders
Many types of sleep-related respiratory dysrhythmia have been noted in association with neurologic illnesses [87–89] (Fig. 41.9). The most common types are sleep apnea and sleep hypopnea.


Fig. 41.9
Schematic diagram to show different types of breathing patterns in neurologic illnesses. a Normal breathing pattern. b Upper airway obstructive apnea. c Central apnea. d Mixed apnea (initial central followed by obstructive apnea). e Paradoxical breathing. f Cheyne–Stokes breathing. g Cheyne–Stokes variant pattern. h Inspiratory gasp. i Dysrhythmic breathing. j Biot’s breathing (a special type of ataxic breathing characterized by 2–3 breaths of nearly equal volume followed by long period of apnea). k Ataxic breathing. l Apneustic breathing
Sleep Apnea
Three types of sleep apnea have been noted [87–90]: central, upper airway obstructive, and mixed. Normal individuals may experience a few episodes of sleep apnea, particularly central apnea, at the onset of NREM sleep and during REM sleep. To be of pathologic significance, the sleep apnea should last at least 10 s and the apnea index (number of apneas per hour of sleep) should be at least 5. In the American Academy of Sleep Medicine (AASM) scoring criteria [89], in addition to duration of 10 s, apnea is scored when the peak amplitude drops by 90 % or more of the baseline, and this amplitude reduction must last for at least 90 % of the event’s duration.
Cessation of airflow with no respiratory effort constitutes central apnea. During this period, there is no diaphragmatic and intercostal muscle activity or air exchange through the nose or mouth. Upper airway obstructive sleep apnea (OSA) is manifested by the absence of air exchange through the nose or mouth but persistence of diaphragmatic and intercostal muscle activity.
During mixed apnea, initially airflow ceases, as does respiratory effort (central apnea); this is followed by a period of upper airway OSA. On rare occasions, this pattern may be reversed, resulting in an initial period of OSA followed by central apnea (Fig. 41.10).


Fig. 41.10
Polysomnographic recording in a patient with narcolepsy and sleep apnea showing the electroencephalogram (EEG; top eight channels); vertical (EOGV) and horizontal (EOGH) electrooculograms; mentalis (MENT), submental (SUBMENT), orbicularis oris (ORIS), left (L) and right (R) alae nasi, and intercostal (INT) electromyogram (EMG); nasal and oral airflow; and abdominal pneumogram (ABD PNEUMO). Note unusual type of mixed apnea (initial obstructive apnea for a period of 14 s followed by central apnea for a period of 8 s) during REM sleep
Sleep-Related Hypopnea
Sleep-related hypopnea is manifested by decreasing airflow at the mouth and nose and decreased thoracoabdominal movement causing a reduction in tidal volume. Until recently, there was no standard definition of hypopnea; however, in the 2007 AASM scoring criteria [89], the recommended definition for hypopnea is a reduction of nasal pressure signal excursion (or that of the alternative airflow sensor) by 30 % or more of the baseline amplitude lasting for a period of at least 10 s and accompanied by a 3 % or more desaturation from the pre-event baseline or the event is associated with an arousal. Furthermore, at least 90 % of the event’s duration must meet the amplitude reduction criteria for hypopnea. An alternative suggestion in the subsequent slight modification (the version 2.1 update) of the same manual is a reduction of the amplitude excursion in the nasal pressure signal (or that of the alternative airflow sensor) by 30 % or more of the baseline lasting for at least 10 s and accompanied by oxygen desaturation of 4 % or more from the pre-event baseline. This amplitude reduction must be present for at least 90 % of the event’s duration. An apnea-hypopnea index (AHI; defined as the number of apneas plus hypopneas per hour of sleep) of 5 or less is considered normal. The respiratory disturbance index (RDI), a term often incorrectly used interchangeably with AHI, includes respiratory effort-related arousals in addition to apneas and hypopneas per hour of sleep. Most investigators consider an AHI or RDI of 10 or more to be clinically significant.
Sleep-related apneas and hypopneas in neurologic diseases are secondary sleep apnea syndromes, in contrast to primary OSA syndrome, in which no cause except for some minor deviations of the upper airway anatomic configuration is found to account for the appearance of apnea. The neurologic illness may be aggravated by the secondary sleep apnea because of the adverse effects of sleep-induced hypoxemia and hypercapnia and repeated arousals with sleep fragmentation. In long-standing cases, there may be pulmonary hypertension, congestive cardiac failure, and other manifestations of chronic sleep deprivation.
Paradoxical Breathing
The thorax and abdomen move in opposite directions during paradoxical breathing, indicating increased upper airway resistance. In upper airway resistance syndrome, this may be noted without any change in oronasal airflow; in OSA, however, paradoxical breathing is accompanied by the reduction or absence of oronasal airflow.
Cheyne–Stokes and Cheyne–Stokes Variant Patterns of Breathing
Cheyne–Stokes breathing (CSB) is a special type of central apnea manifested as cyclic changes in breathing with a crescendo-decrescendo sequence separated by central apneas (see Fig. 41.11) [91–94]. The Cheyne–Stokes variant pattern of breathing is distinguished by the substitution of hypopneas for apneas [19, 21]. In neurologic disorders, the Cheyne–Stokes type of breathing is mostly noted in bilateral cerebral hemispheric lesions [12, 13] and it worsens during sleep, whereas Cheyne–Stokes variant patterns of breathing may also be noted in brain stem lesions, in addition to bilateral cerebral hemispheric disease. In the AASM scoring manual [89], CSB is scored if there are at least 3 consecutive cycles of cyclical crescendo-decrescendo change in breathing amplitude accompanied by at least one of the following: five or more central apneas and hypopneas per hour of sleep; and a cyclic crescendo-decrescendo change in breathing amplitude and duration of at least 10 consecutive minutes. The cycle length is most commonly in the range of 60 s but must be at least 45 s in duration. The arousals typically occur in the middle of the hyperventilation cycle. This breathing pattern is most prominently seen in NREM sleep, particularly stages 1 and 2 and attenuates or disappears during REM sleep. Besides neurologic lesions, this pattern of periodic breathing is noted in patients with severe congestive cardiac failure and as an adverse effect of opiate intake.


Fig. 41.11
A 500-second excerpt showing the classic Cheyne–Stokes breathing (CSB) crescendo-decrescendo pattern (arrows) from an overnight polysomnographic (PSG) recording of a 69-year-old man. The presence of CSB throughout most of the nonrapid eye movement sleep (with marked decrement or absence during rapid eye movement sleep) in this patient with a history of hypertension and excessive daytime sleepiness suggests occult left ventricular failure. ECG electrocardiogram; EEG electroencephalogram; SaO2 oxygen saturation; Left Eye and Right Eye: Electro-oculograms; Chin: Electromyogram from mental and submental muscles; Left and Right Tibialis: Electromyograms from these muscles; Oronasal Flow: Respiratory flow from thermisters; Thorax and Abdomen: Respiratory effort
Dysrhythmic Breathing
Dysrhythmic breathing [92, 94] is characterized by nonrhythmic respiration of irregular rate, rhythm, and amplitude during wakefulness with or without O2 desaturation that becomes worse during sleep. Dysrhythmic breathing may result from an abnormality in the automatic respiratory pattern generator in the brain stem and is commonly seen in multiple system atrophy.
Apneustic Breathing
Apneustic breathing is characterized by prolonged inspiration with an increase in the ratio of inspiratory to expiratory time [95–97]. This type of breathing may result from a neurologic lesion in the caudal pons that disconnects the so-called apneustic center in the lower pons from the pneumotaxic center (parabrachial and Kölliker–Fuse nuclei) in the upper pons in association with vagotomy [95–97].
Inspiratory Gasp
Ataxic Breathing
Biot’s Breathing
Biot’s breathing is a special type of cluster breathing (ataxic breathing) characterized by two to three breaths of nearly equal volume separated by long periods of apnea [97]. This is really a variant of ataxic or cluster breathing and may be found in patients with medullary lesions.
Other Abnormal Breathing Patterns
The following abnormal breathing patterns have also been noted in neurologic disorders, particularly in patients with Shy–Drager syndrome (multiple system atrophy [MSA]) [87].
Nocturnal stridor causing severe inspiratory breathing difficulty,
Periodic central apnea in the erect position accompanied by postural fall of blood pressure in Shy–Drager syndrome (Fig. 41.12a and b) [99],
Fig. 41.12
Periodic central apnea in erect posture in a case of Shy–Drager Syndrome. a supine posture. b erect posture
Prolonged periods of central apnea accompanied by mild O2 desaturation in relaxed wakefulness, as if the respiratory centers “forgot” to breathe [87, 91, 94],
Transient occlusion of the upper airway or transient uncoupling of intercostal and diaphragmatic muscle activity [94],
Transient sudden respiratory arrest,
Catathrenia (bradypnea and groaning), characterized by prolonged expiration with the characteristic groaning noise. This is seen mostly in REM sleep but has also been noted in NREM sleep. This may be mistaken for a central apnea but is really not an apnea, and there is no oxygen desaturation during the episode [100] (Fig. 41.13). It is included in ICSD-3 under sleep-related breathing disorder section as an isolated symptom and normal variant. The etiology and mechanism are at present unknown.
Fig. 41.13
Catathrenia (expiratory groan). A polysomnographic segment of a 120-s epoch showing the electroencephalogram (EEG: C3-A2, O1-A2, C4-A1, O2-A1); left and right eye movements (LOC-A2; ROC-A1); chin, left tibialis (LT.TIBI), and right tibialis (RT. TIBI) electromyograms (EMGs); snoring (SNORE); electrocardiogram (EKG); oronasal airflow (ORONAS); thoracic (THORAX) and abdominal (ABDM) effort channels; and oxygen saturation (SaO2). Note prolonged expiration in the flow and effort channels followed by arousals without oxygen desaturation in stage 2 NREM sleep. Reproduced with the permission from Siddiqui et al. [100]
Sleep-Related Hypoventilation
Finally, sleep-related hypoventilation [101], a type of respiratory dysrhythmia without any apnea or hypopnea, is seen commonly in neuromuscular and intrinsic pulmonary and thoracic restrictive disorders and sometimes in brain stem lesions. Sleep-related hypoventilation is characterized by an increase in the partial pressure of arterial carbon dioxide (Paco 2) of 10 mm Hg above the supine awake values during sleep. An abnormal rise in Paco 2 immediately on awakening from sleep is suggestive of sleep hypoventilation. Persistent oxygen desaturation is not sufficient to document hypoventilation.
Mechanism of Respiratory Dysrhythmias in Neurologic Disease
Several mechanisms may be responsible for the respiratory abnormalities in sleep associated with neurologic disorders [87, 91].
- 1
Direct involvement causing structural alterations of the medullary respiratory neurons (automatic or metabolic respiratory controlling system) may result in apnea or hypopnea during NREM and REM sleep. During REM sleep, this problem may be aggravated because of the additional complicating factor of oropharyngeal or other upper airway muscle hypotonia contributing to upper airway OSA.
- 2
Involvement of the voluntary respiratory control system causes respiratory dysfunction during wakefulness and may give rise to respiratory apraxia.
- 3
Functional or neurochemical alteration of the respiratory neurons may cause respiratory dysrhythmia.
- 4
Interference with the afferent inputs to the medullary respiratory neurons (e.g., compromise of the peripheral chemoreceptors located in the vagal and glossopharyngeal nerve endings), supramedullary pathways, and central chemoreceptors in the ventrolateral medulla may cause abnormal breathing.
- 5
Direct involvement of the efferent mechanism through respiratory muscle weakness may result from either direct involvement of the muscles, as in myopathies, or involvement of the lower motor neurons to the respiratory muscles. In patients with weakness of the principal respiratory and the accessory respiratory muscles, the central respiratory neurons may increase their rate of firing or recruit additional respiratory neurons during wakefulness to maintain ventilation at a level adequate to drive the weak respiratory muscles. Because of the normal vulnerability of the respiratory neurons during sleep, the central respiratory neurons may not be able to participate in such compensatory mechanisms during sleep in patients with respiratory muscle weakness. Ventilatory problems may thus be aggravated, causing more severe hypoventilation and even apnea during sleep. In addition, weakness of the upper airway muscles, which in fact are respiratory muscles and receive phasic inspiratory drive from the respiratory neurons in the brain stem, may cause obstructive apnea.
Sleep and Breathing Disorders Secondary to Somatic Neurologic Illness
Neurologic disorders may affect sleep/wake-generating neurons, causing profound sleep disturbances that may include insomnia, hypersomnia, parasomnia, circadian rhythm disorders, and abnormal movements in sleep at night. An adverse interaction between neurologic illness and sleep dysfunction exists. The sleep disturbances may adversely affect the natural course of the neurologic illness. Sleep dysfunction may result from central or peripheral somatic or autonomic neurologic disorders.
A complaint of insomnia may be related to sleep-onset or maintenance difficulties. Insufficient or fragmented night sleep may result in impaired quality of sleep, fatigue, muscle aches and pains, and poor attention and concentration as well as irritability, anxiety, depression, and impairment of daytime function with daytime somnolence. Most of the neurologic disorders cause hypersomnia, but sometimes insomnia is the predominant complaint [102–109]; an important but rare example, fatal familial insomnia (FFI), is described later in this chapter.
Hypersomnia is generally noted in patients with sleep-related respiratory dysrhythmias. Hypersomnia includes excessive daytime sleepiness (EDS) and irresistible sleep attacks. Associated complaints may include daytime fatigue, lack of concentration, impaired motor skills, morning headaches, and absence of symptom relief from additional sleep. In acute neurologic disorders, the clinical features of neurologic dysfunction may overshadow the sleep and sleep-related respiratory problems [91]. Furthermore, many patients with acute neurologic disorders are actually in stupor or coma. Neurologic lesions may disrupt the sleep architecture, for example, altering the percentage of different sleep stages, increasing awakenings, or causing sleep stage shifts. In addition, sleep apnea (which may occur in various neurologic diseases), intrusion of abnormal movements in sleep, and repeated seizures may disrupt the morphology of sleep and sleep stages. Sleep disturbances may impair memory, cognition, or behavior, or cause cardiopulmonary changes secondary to repeated hypoxemia. These effects, secondary to sleep disturbance, can aggravate the primary neurologic condition. Neurologic causes of hypersomnia have been described in Chap. 3.
The parasomnia (excessive motor activity and abnormal behavior intruding during sleep) most commonly noted in neurologic illnesses is REM sleep behavior disorder (RBD). This is characterized by intense motor activity related to dream-enacting behavior and absence of muscle atonia during REM sleep (see Chaps. 49 and 50). It has been suggested that in the setting of degenerative dementia or parkinsonism, RBD is a manifestation of evolving synucleinopathies (e.g., Parkinson’s disease [PD], MSA, and diffuse Lewy body disease [DLBD] with dementia) but is rare in tauopathies (e.g., Alzheimer’s disease [AD]) [105, 108]. Patients with RBD generally do not complain of EDS, and the multiple sleep latency test (MSLT) rarely documents increased somnolence. There is potential for injury to self and others in patients with RBD, and therefore, early recognition and treatment are very important. Circadian sleep-wake rhythm disturbances are noted in some neurologic disorders; most prominently, AD may present as a cyclic agitation syndrome [110]. An excessive amount of nocturnal motor activity may be related to the primary neurologic disease (e.g., dystonia in patients with torsion dystonia and nocturnal frontal lobe epilepsy).
Clinical Manifestations
The clinical manifestations of sleep and breathing disorders in chronic neurologic illnesses may be divided into specific and general features [87, 91]. The specific manifestations depend on the nature of the neurologic deficit. The general features that are relevant to the diagnosis of sleep-related hypoventilation and apnea include EDS, fatigue, early morning headache, unexplained pedal edema, disturbed nocturnal sleep, intellectual deterioration, personality changes, and, in men, impotence. Breathlessness is generally not an important feature of CNS disorders except those illnesses that affect the lower motor neurons to the respiratory muscles. The general symptoms of daytime fatigue, somnolence, and morning headache may be related to frequent arousals at night secondary to repeated apnea or hypopnea and carbon dioxide retention [108]. In patients with neurologic disorders, it is very important to recognize alveolar hypoventilation during sleep because assisted ventilation at night improves the symptoms and protects patients from fatal apnea during sleep. Furthermore, such treatment may prevent the development of serious complications resulting from episodic or prolonged hypoxemia, hypercapnia, and respiratory acidosis in sleep, complications that may include pulmonary hypertension, cor pulmonale, congestive cardiac failure, and occasionally cardiac arrhythmias. Occasionally, neurologic disorders may cause an inversion of the sleep-wake rhythm that is manifested by excessive somnolence during the day and insomnia with agitation during the night [103, 108–110].
To make a clinical diagnosis of sleep disorders or sleep-related breathing disorders (SRBDs), a careful history—from the patient and the caregiver—and a physical examination are essential.
Mechanisms of Sleep Disturbances
Neurologic disorders can be metabolic or structural (e.g., head injury, tumor, infection, toxic-metabolic brain dysfunction, vascular and degenerative CNS disease, headache from any cause, painful peripheral neuropathy, or other neuromuscular disorder). The following are the suggested mechanisms of the sleep disturbances associated with neurologic disorders [87, 91, 105, 108]:
- 1.
Direct involvement of the hypnogenic neurons. Hypofunction of the hypothalamic VLPO neurons or the lower brain stem hypnogenic neurons in the region of the NTS and dysfunction of the thalamus may alter the balance between the waking and the sleeping brain, causing wakefulness or sleeplessness. Similarly, a disorder of the posterior hypothalamic, ARAS, or other brain regions responsible for waking and alertness causes hypersomnolence.
- 2.
Indirect mechanisms associated with the disorder, such as pain, confusional episodes, changes in the sensorimotor system, and movement disorders, can interfere with sleep.
- 3.
Medications used to treat neurologic illnesses (e.g., anticonvulsants, antidepressants, dopamine agonists, anticholinergics, hypnotics, and sedatives) may have a direct effect on sleep and breathing.
- 4.
Neurologic diseases (e.g., hyperkinetic movement disorders and Rett syndrome) may change the neurochemical environment of the sleep-generating and sleep-promoting neurons [107].
- 5.
Comorbid depression or anxiety in neurologic illnesses may disrupt sleep.
- 6.
Certain neurologic disorders may alter circadian rhythm in the suprachiasmatic nuclei (e.g., dementia, PD, and traumatic brain injury), causing insomnia.
- 7.
Finally, neurologic disorders may cause sleep-disordered breathing (e.g., MSA, DLBD, and other neurodegenerative and neuromuscular disorders), resulting in sleep fragmentation and insomnia, and sleep apnea syndrome.
Sleep and Breathing Disturbances in Central Nervous System Disorders
Alzheimer’s Disease and Related Dementias
Dementia is characterized by progressive deterioration of memory and cognition (as assessed by mental status and neuropsychologic testing), followed by language dysfunction, hallucinations, other psychotic features, depression, and sleep disturbances. In the advanced stage of the illness, the patient becomes bedridden, mute, and incontinent. Sleep dysfunction with or without abnormal motor activity during sleep is increasingly recognized in patients with irreversible chronic dementing illness. It has been estimated that approximately 10 % of the adult population over the age of 65 years suffer from some kind of dementing illness. The Delphi study in 2005 [111] estimated that there would be 24.3 million people with dementia in the world in 2001, and this was predicted to rise to 81.1 million by 2040. The same panel of experts in a systematic review and metanalysis in 2013 [112] estimated that 35.6 million people lived with dementia worldwide in 2010 with numbers expected to rise to 115.4 million in 2050. However, the Rotterdam study findings published in 2012 [113] provide an optimistic trend that the age-specific dementia incidence has declined between 1990 and 2005 as the population has lived progressively longer, probably as a result of increased use of treatment to minimize vascular risk factors and increased years of education. This observation was reinforced by recent report of robust evidence provided by Satizabal et al. [114] of declining incidence of dementia over three decades in the Framingham Heart Study participants. AD is the most common cause of chronic dementia, accounting for at least 60 % of all cases. Other conditions include DLBD, accounting for at least 15–20 % of the cases; PD with dementia and frontotemporal dementia (FTD) in about 10 %; and corticobasal degeneration (CBD), progressive supranuclear palsy (PSP), multi-infarct or vascular dementia, Huntington’s disease (HD), Creutzfeldt–Jakob disease (CJD), and FFI (described later in the chapter), accounting for the rest of the cases.
Dementia can be classified according to location or according to molecular neurobiologic abnormalities (Box 41.1). In terms of location, dementia is classified into cortical dementia, consisting of AD, FTD, and vascular dementia; subcortical dementia, consisting of PD with dementia, PSP, and HD; and mixed cortical-subcortical dementia, including DLBD, CBD, CJD, and FFI. Most of the degenerative dementing illnesses are proteinopathies due to excessive protein misfolding and intracellular protein aggregation [108]. They are mainly classified into tauopathies, synucleinopathies, prion diseases, and polyglutamine disease. Tau proteins belong to the family of microtubule-associated proteins involved in maintaining the cell shape and serve as tracts for axon transport. The main tauopathies include AD, PSP, CBD, argyrophilic grain disease, Pick’s disease, and FTD with parkinsonism associated with chromosome 17. Alpha-synuclein is a presynaptic protein that helps transport dopamine-laden vessels from the cell body to the synaptic cleft. Synucleinopathies are a group of disorders with abnormal deposition of α-synuclein in the cytoplasm of neurons or glial cells and extracellular deposits of amyloid. The main synucleinopathies include PD, DLBD, and MSA, including Shy–Drager syndrome, striatonigral degeneration, and sporadic olivopontocerebellar atrophy.
Box 41.1 Classification of Dementia
According to Location
Cortical dementia
Alzheimer’s disease,
Frontotemporal dementia,
Vascular dementia.
Subcortical dementia
Parkinson’s disease,
Progressive supranuclear palsy,
Huntington’s disease.
Mixed cortical-subcortical dementia
Diffuse Lewy body disease,
Corticobasal degeneration,
Creutzfeldt–Jakob disease,
Fatal familial insomnia.
According To Molecular Neurobiology
Tauopathies
Alzheimer’s disease,
Frontotemporal dementia,
Progressive supranuclear palsy,
Corticobasal degeneration.
Synucleinopathies
Diffuse Lewy body disease,
Parkinson’s disease,
Multiple system atrophy (Shy–Drager syndrome).
Prion diseases
Fatal familial insomnia,
Creutzfeldt–Jakob disease,
Polyglutamine disorder,
Huntington’s disease.
Types of Sleep Disturbances in Dementia
The major sleep disturbances in dementing illness include insomnia, hypersomnia, circadian sleep-wake rhythm disorders, excessive nocturnal motor activity, “sundowning,” and respiratory dysrhythmias. Circadian sleep-wake rhythm disturbances are noted, most prominently in AD, and may present as a cyclic agitation syndrome that is popularly known as sundowning syndrome. Commonly encountered excessive nocturnal motor activity that may occasionally cause sleep disturbance to the patient but very often causes sleep disturbance to the bed partner includes periodic limb movements in sleep (PLMS), which may be noted in many dementing illnesses. Respiratory dysrhythmias and loud snoring (see further on) during sleep occur in some of these conditions, particularly in AD, PD, and DLBD.
Sleep Dysfunction in Alzheimer’s Disease
Diagnostic criteria for AD
AD or Alzheimer’s dementia is characterized by progressive intellectual deterioration occurring in middle or later life associated with characteristic neuropathologic findings, including cerebral cortical atrophy and neuronal loss in the nucleus basalis of Meynert. There are also alterations in the forebrain cholinergic and noradrenergic systems [109]. Sleep disturbances in AD may be related partly to the severity of the loss of the cholinergic neurons in the basal forebrain regions, as well as to changes in the brain stem aminergic systems. Since the introduction of the criteria for clinical diagnosis of AD developed by the National Institute of Neurological and Communicative Disorders and Stroke–Alzheimer’s Disease and Related Diseases Association (NINCDS-ADRDA) work group in 1984 [115], there have been significant advances in the development of reliable biomarkers for AD based on structural magnetic resonance imaging (MRI), molecular neuroimaging with positron-emission tomography (PET), and cerebrospinal fluid (CSF) analysis. Based on these advances, in 2011 the National Institute on Aging and the Alzheimer’s Association (NIA-AA) revised the criteria for diagnosing AD (Table 41.1) [116–119]. In the revised criteria, the terminology was changed to Alzheimer’s dementia rather than Alzheimer’s disease. A diagnosis of dementia requires deficits in two of the following five features: episodic memory, executive function, visuospatial performance, language, and personality/behavior including activities of daily living (ADL) [117–119]. The new diagnostic criteria recognize that AD exists along a continuum and include three categories: preclinical (stages 1, 2, 3), mild cognitive impairment (MCI) due to AD, and AD dementia (probable, possible, unlikely). The biomarkers in the revised criteria include markers for amyloid (e.g., cerebrospinal fluid [CSF], low amyloid-β [A β 42], and abnormal amyloid PET imaging) and neuronal injury (e.g., increased levels of CSF tau protein and hippocampal atrophy on structural magnetic resonance imaging [MRI] or bilateral parietal hypometabolism on PET scan). The NIA-AA criteria incorporated many of the criteria proposed by the International Work Group (IWG) initially devised in 2007 and later revised in 2010 (Box 41.2) [116–119]. The IWG criteria recognize four diagnostic groups [119]: asymptomatic AD at risk stage showing positive biomarkers; preclinical early-onset AD with an autosomal dominant mutation (about 5 % of familial AD cases); prodromal AD with episodic memory loss and presence of a biomarker for AD; and AD dementia with impaired ADL (Table 41.1). In addition, diagnostic criteria have been established for non-AD dementias (e.g., FTD, DLBD with dementia, CBD, and vascular dementia). Many of these entities may fulfill the original NINCDS-ADRDA criteria, but the diagnostic accuracy of the original criteria ranges from 65 % to 96 %, and the specificity ranges from 23 % to 88 %. The new diagnostic criteria, however, need validation studies to optimize their sensitivity and specificity. Furthermore, current biomarkers have limitations (e.g., invasive or expensive). Recently, a group of investigators predicted phenoconversion of some elderly healthy individuals to mild cognitive impairment (MCI) or AD based on lower plasma levels of a set of plasma phospholipids [120]. Confirmation and replication of these findings will simplify preclinical diagnosis of AD and will stimulate research to prevent or halt the progression of the disease.
Category | Subcategory | Symptoms/Signs | Biomarkers |
---|---|---|---|
Preclinical | Stage 1 | Asymptomatic | Amyloid beta (Aβ) (CSF or PET scan) |
Stage 2 | Asymptomatic | Aβ + neuronal injury | |
Stage 3 | Asymptomatic | Aβ + neuronal injury | |
Mild cognitive impairment | Intermediate likelihood of conversion to AD | Impaired episodic memory | Aβ + neuronal injury |
High likelihood | Other cognitive impairment | Aβ + neuronal injury | |
Unlikely | Mild functional impairment | Biomarker negative | |
AD dementia | Probable | Amnestic; impaired learning | Aβ + neuronal injury |
Nonamnestic executive dysfunction | Aβ + neuronal injury | ||
Possible | Meets clinical criteria for dementia | Aβ + neuronal injury | |
Unlikely due to AD | Meets clinical criteria for typical or atypical AD dementia | Biomarkers negative |
Box 41.2 International Work Group (IWG) Criteria for the Clinical Diagnosis of Alzheimer’s Disease (AD)
Adapted from Dubois et al. [116].
Probable AD Criteria
Category A and one or more supportive features in B, C, D, or E
Core Diagnostic Criteria
- (A)
Presence of an early and significant episodic memory impairment with gradual and progressive alteration in memory function over the previous 6 months or more associated with the objective evidence of impaired episodic memory.
Supportive Features
- (B)
Presence of medial temporal lobe atrophy on MRI.
- (C)
Abnormal cerebrospinal fluid biomarker with low amyloid-β (1-42) concentrations with increased tau concentrations.
- (D)
Specific pattern on functional neuroimaging with PET showing reduced glucose metabolism in temporal parietal regions bilaterally.
- (E)
Proven AD autosomal dominant mutation within the immediate family.
Sleep dysfunction in AD
Sleep dysfunction in AD may occur even in the early stage but is more common and severe in advanced stages. In addition to sundowning, these patients often sleep early in the evening, waking up frequently and staying awake most of the night. Their sleep is fragmented and fractionated throughout a 24-h period. The patients remain somnolent most of the time and bedridden in the advanced stages of the illness.
Sleep dysfunction in AD is very common and includes insomnia and sleep apnea syndrome with sleep fragmentation, hypersomnia related to intrinsic disease process, comorbid medical or psychiatric disorders or ingestion of medications, circadian rhythm sleep disorders including inversion of sleep rhythm and “sun downing,” excessive nocturnal motor activity including occasional REM behavior disorder (Box 41.3).
Box 41.3 Summary of Sleep Dysfunction in Alzheimer’s Disease
May occur in the early stage,
More common and severe in advanced stages,
Sleep onset in the early evening; waking up frequently or staying awake most of the night,
Sleep architectural changes, including decreased sleep efficiency, reduced slow-wave sleep with eventual disappearance as the disease advances, increased awakenings after sleep onset, and reduced REM sleep in later stages,
“Sundowning,”
Fragmented and fractionated sleep throughout the 24-hour period with increased daytime napping,
Sleep apnea: 33–53 %; more with APOE4 allele, possibly also with MAO-A 4 repeat allele,
Visual hallucinations with nocturnal agitations.
In advanced stage, somnolent most of the time.
Sleep disorders in AD may increase cognitive and behavioral dysfunction. Such sleep disorders may arise directly from the disease itself, as a consequence of the degeneration of the brain stem and other centers that regulate sleep [31, 109], or indirectly from changes in sleep associated with aging (see Chap. 51) and comorbid conditions or medications (see Chap. 47). Sleep disorders can have a number of undesirable consequences, including increasing cardiovascular and even cerebrovascular morbidity as well as impairing daytime alertness and functioning [91, 105, 108].
A number of studies have examined the differences between demented patients and normal elderly individuals and have demonstrated higher prevalence of sleep apnea and poorer sleep quality when patients are compared to age-matched controls [121–131]. Although the results vary somewhat from study to study, these investigations have shown deterioration of sleep parameters, including reduced total length of sleep, decreased REM and slow-wave sleep, loss of phasic components (spindles and K complexes) of NREM sleep, and sleep-wake rhythm disturbances, in demented patients. Montplaisir et al. [128] documented EEG slowing during both wakefulness and REM sleep in AD patients. The authors suggested that degeneration of the nucleus basalis of Meynert, which is the main source of cholinergic input to the cerebral cortex, may be responsible for EEG slowing and REM sleep changes. This pattern of disorder is different from that of depressed elderly patients, who most clearly show poor sleep maintenance, often with increased REM sleep [128]. Most of the studies, however, have not used current diagnostic criteria for dementia and have lumped together patients with different forms of it. When more accurate diagnostic groupings were made, similar results were found for AD patients, usually defined by clinical course. Some studies have shown a clear association between greater sleep disturbance and impaired mental functioning or severity of dementia [132–136].
Some of the inconsistencies noted in the sleep architecture in AD patients may be related to the fact that in many studies, mild, moderate, and severe AD patients were grouped together and not necessarily analyzed separately. Another point to remember is that it is often difficult to separate the effects of the disease on sleep from the effects of medication, PLMS or sleep apneas, which are common in elderly patients. Additionally, sleep architectural alterations noted during overnight laboratory sleep studies may be partly environmentally determined, as AD patients may become confused, displaying features of sundowning, in the artificial and foreign environment of the laboratory.
Vitiello et al. [133, 134] and others [123, 124, 135–138] reported sleep disturbances in AD associated with a decrease in slow-wave sleep and an increase in nighttime awakenings. In a study of 45 control subjects and 44 mild AD patients, Vitiello’s group [139] confirmed their previous findings of disturbed sleep-wake patterns in AD patients, but the phenomenon of sleep disturbances was not diagnostically useful for discriminating between those with a mild stage of AD and control subjects.
A meta-analysis by Benca et al. [132] clearly showed significant sleep disturbances in dementia. Reduced sleep efficiency, increased stage 1 NREM sleep, and increased number of awakenings are some of the prominent findings noted in the various studies [127, 128, 131–144]. In some studies, there is a relationship between the severity of sleep disturbance and the severity of dementia [139]. Contradictory findings have been noted regarding decrement of slow-wave sleep. Reduction of sleep spindles is noted by Prinz et al. [135] as well as by Montplaisir et al. [145]. REM sleep abnormalities have given inconsistent results (decreased in some studies [131, 139] but not in others [142]), and this discrepancy may have been related to the degree of severity of dementia. It should be kept in mind that more severe sleep disturbances have been noted in depression than in dementia, and sometimes differentiating these two conditions, particularly in the early stage of AD, may be difficult. Other sleep architectural changes in AD include sleep-wake cycle [145] and sleep rhythm [146, 147] and disturbances and alterations in physiologic delta waves [148]. These sleep quality changes may cause EDS [148, 149].
Sleep may be disturbed early in the disease process, and sleep disturbances are noted even in the presence of mild cognitive impairment [139, 150–153]. It has been recently shown that sleep loss may even precede AD symptoms by many years similar to CSF biomarkers [154–156]. It has been suggested that sleep disturbances and cognitive dysfunction are positively correlated in AD—that is, sleep disturbances increase with severity of disease process [157]. Disruption in sleep-wake patterns, circadian rhythmicity, increased amount and frequency of nighttime wakefulness, and reduction of slow-wave sleep occur at the early stages of AD and worsen with disease progression. In later stages of AD, there is a reduction of REM sleep, increased REM latency, and alteration of the circadian rhythm resulting in daytime sleepiness. The daytime sleep, however, consists essentially of NREM stages 1 and 2 and does not compensate effectively for the loss of slow-wave sleep and REM sleep. Thus, daytime napping and somnolence increase as the disease progresses.
The phenomenon of sundowning is noted in many AD patients, contributing to sleep disturbance. Sundowning can be described as cyclic nocturnal agitation syndrome [110, 151–153] with inversion of sleep schedule (wakefulness at night and somnolence in the daytime). It is a predictor of faster cognitive decline [157], is most likely related to the severity of dementia, and remains a common cause of institutionalization in AD patients. Factors such as going to bed early, increased use of sedatives, advanced cognitive impairment, associated medical conditions, and circadian rhythm disturbances may all contribute to sundowning. Circadian rhythm disturbances are frequently seen and pronounced in AD [110, 152, 153, 158–163]. It has been suggested that an alteration in the biologic clock in the SCN and the pineal gland is considered to be the biologic basis for the circadian rhythm disturbances in AD. Wu and Swaab [158] found disruption of pineal melatonin secretion and pineal clock gene oscillations in AD patients. They noted even in the earliest AD stage a functional disruption of the SCN as manifested by decreased vasopressin RNA, a clock-controlled major output of the SCN. The functional disconnection between the SCN and the pineal gland noted in the earliest stage of AD seems to account for the pineal clock gene and melatonin changes accounting for the circadian rhythm disturbances in AD. They also noted decreased melatonin MT1 receptor in the SCN in late-stage AD patients and therefore suggested that in the advanced stages of AD, supplementary treatment with melatonin may not improve the circadian rhythm disturbances. Wu and Swaab [159] previously suggested that circadian disorders, such as sleep-wake cycle disturbances, associated with aging and advanced stages of AD causing disruptive melatonin production and rhythms may result from presumed degeneration of the retina-SCN-pineal axis. They further suggested that reactivation of the circadian system (retina-SCN-pineal pathway) by the use of light therapy and melatonin supplementation to restore the circadian rhythm and relieve clinical circadian disturbances has shown promising results.
However, there are contradictory reports [160, 161]. Dowling et al. [160] tested the effectiveness of time to bright-light therapy given in the morning and early afternoon in 70 institutionalized patients with AD and controls. They did not find any significant differences in actigraphy-based measures of nighttime sleep or daytime wakefulness between the groups. They therefore concluded that one hour of bright-light treatment in patients with AD in the morning or early afternoon did not improve nighttime sleep or daytime wakefulness compared to the control group. In another study, Dowling et al. [161] tested the effect of morning bright-light therapy in 46 AD subjects fulfilling the NINCDS-ADRDA criteria in two nursing home facilities in California. They gave one hour of bright-light exposure (≥2500 lx) to the experimental group and gave usual indoor light (150–200 lx) to the control group. By means of actigraphy, they assessed nighttime sleep efficiency, total sleep time, and number of awakenings. They also determined circadian rhythm parameters from the actigraphy data using core cosinor analysis and nonparametric techniques. They concluded that morning bright-light exposure did not induce an overall improvement in measures of sleep or rest activity in all treated patients compared to control subjects. However, they found that only subjects with the most impaired rest activity rhythm responded significantly and positively to a bright (one hour) light therapy. However, in a more recent study using randomized controlled trial, McCurry et al. [164] found that walking, light exposure, and combination are effective in improving sleep in community-dwelling AD patients. Singer et al. [162] conducted a multicenter, placebo-controlled trial of melatonin for sleep disturbance in 157 individuals with AD recruited by 36 AD centers. They measured nocturnal total sleep time, sleep efficiency, wake time after sleep onset, and day-night sleep ratio during a 2- to 3-week baseline and 2 months of treatment with melatonin. The sleep measures were obtained from the actigraphic data. They did not find any statistically significant differences in objective sleep measures between baseline and treatment for any of the group. Therefore, they concluded that melatonin is not an effective agent for treating sleep disturbances in patients with AD. This observation by Singer et al. [162] agrees with more recent conclusion based on a review of randomized controlled trials by McClury et al. [165] that there is no evidence of benefit from melatonin treatment in AD patients. The results of these studies, therefore, contradict the earlier findings of Mishima et al. [163] of altered melatonin secretion rhythms in patients with AD having disturbed sleep-wake patterns.
Sleep apnea has been observed in approximately 33–53 % of demented patients with probable AD [131, 133, 152, 153, 166–173] (Fig. 41.14). Although sleep apnea may be associated with disease severity, no longitudinal studies have been conducted to determine whether sleep apnea increases the severity of disease in individual patients and whether sleep apnea may be associated with more rapid progression of the disease. Such a deleterious effect of sleep apnea is to be expected, as it is thought to increase the intellectual deficit of demented patients [128, 129]. Because sleep apnea may be treated by a number of modalities, it is possible that therapy may improve behavior and cognitive function. According to Smallwood et al. [131], the incidence of sleep apnea in male AD patients is similar to that in healthy elderly subjects. Reynolds et al. [167] reported a higher prevalence of sleep apnea in female AD patients during the later stage of the illness than in controls. These findings have been confirmed by Mant et al. [168]. The prevalence of sleep apnea is found to vary (70–80 % having an apnea-hypopnea index [AHI] of 5 or more and 38–48 % with an AHI of 20 or more in institutionalized demented patients) [174, 175]. However, in mild-to-moderate AD, the prevalence with AHI of 10 or more is up to 53 % of patients [176]. Bliwise [169] pointed out the high prevalence of comorbid cardiovascular, cerebrovascular, and pulmonary disease in the elderly population in the nursing home and outpatient memory clinics. These disorders may have played a causal role for sleep apnea and dementia. A key genotypic marker of AD is the apolipoprotein E4 (APOE4) allele. In recent years, the demonstration of an association between APOE4-genotype AD and sleep apnea has kindled interest in the possible association between sleep apnea and AD [169–173]. Gehrman et al. [172] suggested sleep apnea or sleep-disordered breathing (SDB) may be related to agitation in AD. These authors recorded sleep for one night and measured agitation with behavioral observations and ratings by nursing staff in 38 patients (29 women, 9 men) in a nursing home population. They found that SDB was very prevalent in this sample and was related to some types of agitation during the day but not in the evening and night. They further suggested that treatment of SDB may decrease agitation in these patients. In a later study by this group [173] utilizing 66 patients with mild-to-moderate AD in a home polysomnographic (PSG) study, the authors observed that the patients with SDB spent less time in REM sleep than those with no SDB, but they did not find any differences in other sleep stages. They concluded that decreased REM sleep may be due to the presence of SDB in AD. They further speculated that treating these patients’ SDB may increase their amount of REM sleep, which may result in improved daytime functioning. Chong et al. [177] randomly assigned 39 community-dwelling elderly patients with mild-to-moderate probable AD with SDB to receive 6 weeks of therapy with continuous positive airway pressure (CPAP) titration or 3 weeks of sham CPAP followed by 3 weeks of therapeutic CPAP. They measured Epworth Sleepiness Scale (ESS) scores at baseline, 3 weeks, and 6 weeks to measure the changes in daytime sleepiness. Their results of reduction of ESS scores after therapeutic but not sham CPAP treatment supported the effectiveness of CPAP in reducing subjective daytime sleepiness in AD patients with SDB. Does CPAP slow cognitive decline in patients with comorbid Alzheimer diseases and sleep apnea? Preliminary studies support improvement or slowing of the progression of cognitive impairment as well as quality of sleep and architecture in AD patients [176, 178–181] although there are limitations [176, 182].


Fig. 41.14
Polysomnographic recording of a patient in an advanced stage of Alzheimer’s disease shows a portion of mixed apnea during stage 2 NREM sleep accompanied by oxygen desaturation. Top four channels represent the electroencephalogram (EEG) (Key: international electrode placement system). Electromyograms (EMG) of mentalis (MENT), submental (SUBMENT), orbicularis (ORIS), sternocleidomastoid (SCM), scalenus anticus (SCAL), alae nasi, and intercostal (INT) muscles are shown. Also shown are nasal and oral airflow, abdominal pneumogram (ABD PNEUMO), and oxygen saturation (SaO2 %). (EOG = electrooculogram.) Reproduced with the permission from Chokroverty [91]
Ancoli-Israel et al. [176] studied 52 men and women with mild-to-moderate AD and OSA who were randomized to either therapeutic CPAP for six weeks or placebo CPAP for three weeks followed by therapeutic CPAP for three weeks in a double-blind placebo-controlled trial. The neuropsychologic test battery showed improvement in some of the cognitive functioning. The same group in a subsequent exploratory study [180] involving 10 patients with mild-to-moderate AD (five patients using CPAP for a mean of 13.3 months and five patients who discontinued CPAP use after the initial six-week randomized clinical trial of CPAP) showed less cognitive decline (based on a neuropsychologic test battery and sleep/mood questionnaires) in the group with sustained CPAP use. Recently, Troussiere et al. [181] in a single-blind, proof-of-concept trial involving 23 patients with mild-to-moderate AD and severe sleep apnea showed that those on CPAP (14 patients) had significantly slower decline in cognitive function over a three-year follow-up period. All these preliminary studies are encouraging but because of many limitations [176, 178, 182] (e.g., small sample size, too short a period of treatment exposure, selection bias, self-reported CPAP use, and failure to adequately consider comorbid medical disorders), there is a need for a prospective randomized controlled trial including larger number of patients and a longer follow-up period.
Pseudodementia in elderly depressed patients presents a frequent diagnostic dilemma in differentiating depression from dementia of the Alzheimer’s type. It has been noted that depressed elderly patients have shortened REM latency and increased REM density; in contrast, AD patients tend to have greater reduction in the amount of REM sleep and lower REM density [183, 184]. These findings, however, are not useful as a predictive indicator on a case-to-case basis.
Hallucinations may occur in AD patients but are noted much less frequently than in DLBD (see later). Sinforiania et al. [185] administered a sleep questionnaire in the presence of a caregiver to 280 patients in order to evaluate the relationship between hallucinations and sleep-wake cycle in patients with early-to-moderate AD. They noted hallucinations, mainly visual, in 12 % of the sample, and 69 % of the hallucinations occurred when the patient was awake. Vivid dreams were reported in 11 %, and violent sleep-related and dream-related behaviors (probable RBD episodes) were noted in 10 % of the subjects. The authors concluded that the higher occurrence of vivid dreams and RBD in AD patients with hallucinations compared with those without hallucinations indicates a potential role of disordered REM sleep in the occurrence of hallucinations in AD. It should be noted that in most of the reports, RBD has not been seen frequently in tauopathies such as AD. Although RBD is rare, REM sleep without atonia may be noted relatively frequently in patients with probable AD [186]. EDS is very common in AD, and in order to assess daytime sleep propensity in a cohort of patients with mild-to-moderate AD, Bonanni et al. [187] studied 20 drug-free AD patients meeting the NINCDS-ADRDA criteria for probable AD and a group of 12 healthy subjects free of dementia as controls. They used multiple sleep latency tests and overnight PSG recordings to evaluate daytime sleepiness. Their findings of significantly reduced daytime sleep latencies indicated an increased sleep propensity during the daytime in patients with mild-to-moderate AD. Park et al. [149] observed that AD patients with excessive daytime napping had more parkinsonian motor signs, suggesting that this subgroup may have an increased propensity for sleepiness resembling PD. The authors cautioned that longitudinal studies with objective measures are needed to determine whether a causal relationship exists between sleepiness and parkinsonism in AD.
The pathogenesis of sleep disturbances in AD is multifactorial, resulting from possible degeneration of neurons regulating sleep-wake cycles, SDB, and disruptive chronobiology. However, no longitudinal studies are currently available correlating sleep apnea with the severity or progression of the disease. Suprachiasmatic nuclei regulating circadian rhythm show degenerative loss of neurons in AD, which may explain inversion of rhythm in many patients. Other factors include normal age-related physiologic changes in sleep, medication effects, increased prevalence of PLMS causing arousal and sleep fragmentation in the elderly AD patients, comorbid RLS, environmental factors (e.g., artificial environment of the institution, laboratory, and nursing homes), and comorbid medical disorders (e.g., congestive cardiac failure, chronic obstructive pulmonary disease, pain from arthritis, nocturia, gastroesophageal reflux disease) or depression. Finally, there may be a genetically increased risk of sleep disruption in AD. Craig et al. [188] surveyed 426 AD patients diagnosed according to standard criteria and performed genotyping of APOE. They found that increased susceptibility to sleep disturbance is associated with genetic variation at the enzyme monoamine oxidase A.
In summary, it is known that sleep dysfunction is common in AD. It is unclear, however, whether a specific set of sleep abnormalities will be found to be associated with AD that are different from those observed in other dementias. Reynolds et al. [124] suggested that sleep dysfunction in AD may be related to the progression of the disease and may cause ongoing deterioration of the alertness, orientation, and cognitive function. Box 41.3 summarizes the sleep dysfunction in AD.
Dementia with Diffuse Lewy Body Disease
DLBD is a neurodegenerative disease characterized by onset of dementia (impaired executive function) within 12 months of onset of motor symptoms of parkinsonism such as akinesia or bradykinesia, postural instability, and rigidity without the characteristic parkinsonian tremor, associated with visuospatial dysfunction, recurrent visual hallucinations, fluctuating cognitive function, and hypersensitivity to neuroleptics. The clinical features of DLBD may be divided into three groups: core, suggestive, and additional features [189]. A recent international consortium on DLBD has resulted in revised criteria [190] for the clinical and pathologic diagnosis, incorporating new information about the core clinical features and improved measures for their assessment. The core features are typically cortical and subcortical cognitive impairment with worse visuospatial and executive dysfunction than AD [189]. In the early stage, the memory dysfunction may be relatively spared. Other core features include recurrent visual hallucinations, parkinsonism, and fluctuating attention. Suggestive features consist of RBD, severe neuroleptic sensitivity, and low dopamine transporter uptake in the basal ganglia on functional neuroimaging. Additional features supporting the diagnosis but occurring less commonly include repeated falls and syncope, transient loss of consciousness, severe autonomic dysfunction, systematized delusions, olfactory and tactile hallucinations, depression, neuroimaging finding of relative preservation of medial temporal lobe structures, reduced occipital activity in functional neuroimaging, EEG slowing, and myocardial scintigraphy showing low uptake [189]. The pathologic criteria include the presence of Lewy bodies in limbic, paralimbic, and neocortical regions in addition to the midbrain substantia nigra, LC, and raphe nuclei. Senile plaques are present in the majority of individuals with DLBD, although neurofibrillary tangles are typically absent.
Studies inquiring about sleep disturbance in DLBD are few given its clinical overlap with AD and PD. Sleep disturbances in DLBD are very common and more prominent than in AD patients. The significant sleep disturbances in DLBD consist of insomnia, daytime hypersomnolence, RBD, sleep apnea, and nocturnal visual hallucinations. Presti et al. [191] in a recent postmortem examination of brains from DLBD patients observed that medullary respiratory neurons involved in respiratory rhythmogenesis and chemosensitivity are affected (decreased neuronal densities by immunocytochemical staining) in DLBD patients accounting for SDB [108, 191] including central apnea and sudden death during sleep and decreased ventilatory response [192] in these patients. Grace et al. [193] made a comparative study of sleep profiles in patients with DLBD and AD. They reported more overall sleep disturbances, more movement disorders in sleep, and more abnormal daytime sleepiness in DLBD patients in comparison with AD patients. RBD is very common in DLBD (present in 50–80 % of DLBD patients) and sometimes may be the initial presentation without other core diagnostic features [194–199]. Boeve and colleagues in a series of studies [40, 200–203] suggested that RBD is a manifestation of an underlying α-synucleinopathy (e.g., PD, DLBD, and MSA) and may be a forerunner or precursor of the disease. Neuropathologic studies by Uchiyama et al. [204] and Boeve et al. [197–203] supported the conclusion that idiopathic RBD may be a preclinical sign of DLBD. Disturbance of the circadian rhythm has been noted and is a potential factor underlying the nocturnal sleep fragmentation and daytime sleepiness in many AD and DLBD patients. Harper et al. [205] studied circadian variation of core body temperature and motor activity in a total of 32 institutionalized patients with probable AD by NINCDS-ADRDA criteria, nine of whom also met pathologic criteria for DLBD and in eight elderly male controls. They noted that patients with a postmortem diagnosis of DLBD manifested greater disturbances of locomotor activity circadian rhythms than patients with AD, which may reflect the greater sleep disturbances seen in this population. Bauman et al. [206] reported EDS with normal CSF hypocretin in 10 DLBD patients.
Sleep Disturbances in Frontotemporal Dementia
FTD is a type of cortical dementia and a tauopathy resembling AD, but there are many features differentiating these two entities. Sleep disturbances are noted in many FTD patients but have not been adequately characterized. The clinical features of FTD include core and supportive features [207]. Core features include insidious onset and progression, early loss of insight, social decline, personal conduct, and emotional blunting with relative preservation of perception, praxis, and memory. Supportive features include perseveration, hyperorality, impersistence, mental inflexibility, decline in personal hygiene, and altered speech and language dysfunction such as echolalia, mutism, reduced speech output, and lack of spontaneity in speech. Physical findings may include akinesia, rigidity, and appearance of primitive reflexes. Laboratory tests may include normal EEG, and neuropsychologic tests show frontal lobe impairment. Computed tomography (CT) and MRI of the brain may show frontal or temporal lobe atrophy.
In a retrospective review of the clinical records, Seeley et al. [208] studied the natural history of the temporal variant of FTD and divided FTD into three stages according to the progression of symptoms. Stage 1 is characterized by either semantic loss characterized by anomia with word-finding difficulties and repetitive speech or an early behavioral syndrome characterized by emotional distance, irritability, and disruption of sleep, appetite, and libido. In stage 2, appearing on an average after 3 years, patients have both semantic and behavioral syndromes. In stage 3, generally 5–7 years after onset, in addition to the above features, patients now have disinhibition, compulsions, impaired face recognition, altered food preference, and weight gain. The authors concluded that the temporal variant of FTD follows a characteristic cognitive and behavioral progression, suggesting early spread from one anterior temporal lobe to the other, and the latest symptoms implicate ventromedial frontal, insular, and inferoposterior temporal regions. However, they caution that precise anatomic correlates need to be confirmed. Liu et al. [209] compared the behavioral features in the frontal and temporal variants of FTD and performed volumetric measurements of the frontal, anterior temporal, and ventromedial frontal cortex and the amygdala in 51 patients with FTD and 20 normal controls as well as 22 patients with AD serving as dementia controls. They found that the group with the frontal variant of FTD showed more anxiety, apathy, and eating disorders, and the group with the temporal variant of FTD showed a higher prevalence of sleep disturbances, than patients with AD. The behavior between the two variants may be differentiated: There is greater apathy in the frontal variant and more sleep disturbances in the temporal variant of FTD. In 13 patients with FTD, actigraphic data suggested possible phase delay [210]. Some patients with FTD may have sleep-wake cycle abnormalities early in the course associated with sleep fragmentation and possible advanced sleep phase syndrome [211]. Coban et el [212] observed reduced orexin A plasma levels in cases of FTD possibly explaining EDS in these patients. Although RBD is rare in tauopathies, Lo Coco et al. [213] described a case of RBD (clinically and polysomnographically) in a case of FTD.
Sleep Dysfunction in Corticobasal Degeneration (CBD)
Corticobasal degeneration (CBD) is a rare tauopathy presenting with an atypical parkinsonian dementia syndrome characterized by asymmetric or unilateral rigidity, action or stimulus-sensitive myoclonus, apraxia, asymmetric or unilateral cortical sensory loss, and alien limb phenomenon, in which the patient cannot recognize his own affected limb, which may be performing apparently purposeful movements not intended by the patient. The pathologic hallmarks include large achromatic neurons and gliosis distributed asymmetrically in discrete frontal or parietal cortical and subcortical regions affected in PSP. Isolated case reports of sleep dysfunction such as insomnia, sleep-related respiratory disorders, PLMS, and RBD or REM without atonia are available [214–217].
Stroke and Sleep-Wake Disorders
Stroke is an acute neurologic deficit resulting from vascular injury to the brain and is the third leading cause of death and disability worldwide. Stroke is responsible for half of all acute neurologic hospital admissions in the USA [218]. Vascular injury could be ischemic (thrombotic or embolic) or hemorrhagic. In this section, we first describe sleep and breathing disorders as well as other sleep-wake dysfunction in cerebral hemispheric and thalamic strokes followed later by those resulting from brain stem stroke.
There are a few scattered reports of sleep complaints after stroke and several reports of SDB after cerebral infarction, but there is a dearth of well-controlled studies of the relationship between sleep disorders and cerebral vascular diseases [218]. Such studies are important from prognostic and therapeutic points of view.
Hemispheric Stroke
Sleep apnea or SDB and stroke
Sleep disruption and sleep complaints resulting from sleep-related breathing dysrhythmias have been reported in many patients with cerebral hemispheric stroke. Sleep apnea, snoring, and stroke are intimately related. Sleep apnea may predispose to stroke, and stroke may predispose to sleep apnea. There is increasing evidence based on case-control, epidemiologic, and laboratory studies that snoring and sleep apnea are risk factors for stroke. Confounding variables that are common risk factors for snoring, sleep apnea, and stroke (e.g., hypertension, cardiac disease, age, body mass index [BMI], smoking, and alcohol consumption) should be considered when attempting to establish relationships among snoring, sleep apnea, and stroke. A history of habitual snoring (established through questionnaire studies and interviews with a bed partner or other family members) is a clear risk factor for stroke. There is an increased frequency of sleep apnea in both infratentorial and supratentorial strokes. Sleep apnea may adversely affect the short-term and long-term outcomes in patients with stroke in terms of both morbidity and mortality. It is important to make the diagnosis of sleep apnea in stroke patients, as there is effective treatment for sleep apnea that can decrease the risk of future stroke.
Case-control and epidemiologic studies have established an association between hypertension and habitual snoring [219–222] and between habitual snoring and stroke [223–227]. The prospective study by Koskenvuo et al. [222], adjusting for other risk factors, found that habitual snorers have a significantly increased risk of new stroke or ischemic heart disease. Neau et al. [228] also found a significantly increased adjusted risk of stroke in habitual snorers. Spriggs et al. [229] found that in addition to increasing the risk for stroke, snoring adversely affected the prognosis after a stroke. In a prospective study, Bassetti et al. [230] used PSG to determine the frequency of habitual snoring and sleep apnea in 36 of 59 subjects within 12 days of acute hemispheric stroke or transient ischemic attack (TIA) and in 19 age- and sex-matched controls. Habitual snoring was reported in 58 % of patients with TIA or stroke, in addition to an increased frequency of sleep apnea in patients with TIA and acute stroke.
There is considerable evidence based on several recent large epidemiologic and many case-control studies showing an independent association between obstructive sleep apnea syndrome (OSAS) and stroke. Sleep apnea has been found in up to 72 % of patients with stroke. The pathogenesis is not clearly known, and most likely is multifactorial, involving sympathetic nervous system hyperactivity, activation of inflammatory molecular pathways, endothelial dysfunction, metabolic dysregulation, abnormal coagulation, dyslipidemia, and insulin resistance [231]. There are several well-established risk factors for the development of stroke, including arterial hypertension, cardiac disease, diabetes mellitus, smoking, and dyslipidemia. Although the evidence of association between OSAS and stroke is strong based on mainly cross-sectional, case-control, and some limited longitudinal studies, large-scale collaborative studies including patients with OSA controlled adequately for potential confounders are needed to evaluate the relationship between OSAS and stroke and the potential interactions between different basic mechanisms (Fig. 41.15). The Sleep Heart Health Study [232] is a cross-sectional study including a sample of 6424 individuals who underwent unattended overnight PSG at home. Sleep apnea was significantly associated with the development of stroke, coronary artery disease, and congestive cardiac failure independent of known cardiovascular risk factors.


Fig. 41.15
Mechanisms of SDB after stroke
As part of the Sleep Heart Health Study in a later report, Redline et al. [233] followed 5422 community-based subjects without stroke for a median of 8.7 years and reported in 2010 that 193 developed ischemic stroke with a significant positive association with AHI in men. Stroke risk was estimated to increase by 6 % for each one-unit increase in AHI in men with mild-to-moderate sleep apnea.
A higher prevalence of OSA has also been shown in patients with TIAs compared with controls by Bassetti and Aldrich [234]. Cross-sectional studies, however, cannot make a definite conclusion about the cause-and-effect relationship, and therefore, prospective longitudinal studies are needed. A prospective cohort study of patients admitted for stroke or TIA by Parra et al. [235] demonstrated a higher prevalence of OSA than in the general population. However, this was contradicted in a small case-control study involving 86 patients with TIA matched for age and sex with controls that showed no significant difference in the severity or prevalence of OSAS between the two groups [236]. A study by Wierzbicka et al. [237] involving 43 patients found a high prevalence of sleep apnea in patients with acute stroke and TIA. The authors suggested that overnight screening for SDB should be routinely performed in every patient admitted with stroke or ischemic attack. Grigg-Damberger [238], in a review article, made a similar suggestion and noted that such screening and treatment for OSAS should be incorporated into stroke prevention programs.
In an important observational cohort study, Yaggi et al. [239] performed PSG in 1022 consecutive patients enrolled in the study and verified subsequent events such as strokes and deaths. Proportional hazards analysis was used to determine the independent effect of OSAS on the composite outcome of stroke or death. At baseline, 697 patients (68 %) had a mean AHI of 35 as compared with 2 in controls. After a median follow-up period of 3.4 years, the OSAS syndrome was associated with stroke or death from any cause with a hazard ratio of 2.24. After adjusting for age, sex, smoking habits, alcohol consumption, BMI, and the presence or absence of diabetes mellitus, hyperlipidemia, atrial fibrillation, and hypertension, OSAS retained its statistically significant association with stroke or death with a hazard ratio of 1.97. The authors concluded that OSAS significantly increases the risk of stroke or death from any cause and the increase is independent of other risk factors. Several other studies confirmed an independent association between OSAS and stroke [240–244]. Harbison et al. [240] performed a prospective, uncontrolled observational study at week 2 and a repeat study in 50 patients at weeks 6–9 following stroke-utilizing sleep studies, modified ranking score, Barthel score, Scandinavian Neurological Stroke Scale, and ESS score. They concluded that SDB improved in the first 6–9 weeks following stroke but remained highly prevalent. They made a surprising observation of the presence of SDB in patients with lacunar stroke. Marin et al. [241] did an observational study to compare the incidence of fatal and nonfatal cardiovascular events, including stroke, in simple snorers. They included patients with untreated OSAS, patients treated with CPAP titration, and healthy men recruited from the general population. The subjects were followed up at least once a year for a minimum of 10.1 years, and CPAP adherence was checked with a built-in meter. The study included 264 healthy men, 377 simple snorers, 403 patients with untreated mild-to-moderate OSAS, 235 with untreated severe disease, and 372 with the disease who were treated with CPAP. They found that patients with untreated severe disease had a higher incidence of both fatal cardiovascular events (deaths from myocardial infarction or stroke) and nonfatal cardiovascular events than did untreated patients with mild-to-moderate disease, simple snorers, patients treated with CPAP, and healthy participants. The authors concluded that in men, severe OSAS significantly increased the risk of fatal and nonfatal cardiovascular events and CPAP treatment reduced this risk. Munoz et al. [242]. performed a prospective 6-year longitudinal population-based study in subjects aged 70–100 years. After adjustment for confounding factors, the authors confirmed that patients with severe OSAS at baseline had an increased risk of developing stroke independent of known confounding factors. Artz et al. [243] also demonstrated after a cross-sectional longitudinal analysis of subjects from the general population that there was a strong association between moderate-to-severe SDB and prevalence of stroke independent of the confounding factors. These authors also provided the first prospective evidence that SDB preceded stroke and may contribute to the development of stroke. In a prospective 10-year follow-up study, Sahlin et al. [244] obtained overnight sleep recordings at a mean of 23 days after the onset of stroke in 132 patients. They found that the risk of death was higher among the 23 patients with obstructive apnea (AHI ≥ 15) than controls (AHI < 15), with an adjusted hazard ratio of 1.76 independent of all the confounding factors. There was no difference in mortality between central sleep apnea (CSA) patients and controls.
Several early studies [245–247] evaluated the role of CPAP in the treatment of patients with OSAS and found significant protection against new vascular events after ischemic stroke. Bassetti et al. [247] found a beneficial effect of CPAP in a small percentage of patients. In contrast, after a randomized controlled trial of CPAP in patients with stroke with an AHI of 30 or more, Hsu et al. [248] found no benefit from CPAP treatment. These authors advocated that CPAP treatment should be used for patients with stroke only if there are symptoms of SDB. Similar to the findings of Bassetti et al. [247], Palombani and Guilleminault [249] found that the majority of stroke patients with OSAS rejected CPAP treatment, and they suggested that better education and support of patients and families, and special training sessions, will be needed to improve adherence in such patients.
Obstructive sleep apnea is the most common form of SDB in stroke victims, and the prevalence in stroke patients exceeds the figure quoted for the general population [218, 236, 250–266]. Bassetti et al. [254] suggested that the presence of OSA should be suspected in men and elderly patients with diabetes mellitus and nighttime onset of TIA or stroke. The increased prevalence of OSAS in patients with TIA and stroke suggests that OSAS does not commonly precede but rather follows the onset of cerebrovascular events [255, 256]. It is notable that acute stroke may aggravate preexisting SDB or even may cause it de novo [255]. Improvement of SDB often occurs in the recovery phase after stroke [254, 256].
Mansukhani et al. [260] from Mayo Clinic in a prospective cohort study included 174 consecutive patients with acute ischemic stroke. Using Berlin Sleep Questionnaire, they identified 105 patients (60.4 %) with a high risk for OSA and seven patients (4 %) with a previous diagnosis of OSA. They found that those with a previous diagnosis of OSA were more likely to die within the first month after stroke and had worse functional outcome. Such comorbid OSA and stroke causing poor functional outcome [252, 257, 261] and increased poststroke mortality [231, 234] had been observed by earlier investigators.
In an important meta-analysis of 29 articles (2343 patients), Johnson and Johnson [262] observed that the percentage of stroke and TIA patients with OSA is up to 72 % (with an AHI of 5 or more) and up to 63 % (with an AHI of 10 or more), and the type of apnea is primarily obstructive (only 7 % had central apnea). They also made the following important observations: Comorbid OSA and stroke are more common in men than women and in older than younger subjects; nocturia may be a predictor and recurrent stroke may be an indicator.
In a recent review of stroke and sleep apnea, Davis et al. [263] cautioned that we may be neglecting a modifiable stroke risk factor. They provide evidence that sleep apnea is an important but under-recognized risk factor for incident and recurrent stroke which may adversely affect stroke recovery and that CPAP titration improves functional outcome after stroke. The authors further state that several preliminary randomized trials of CPAP therapy after stroke suggest diagnosing OSA in stroke patients may be best accomplished with portable devices, but these are less sensitive and specific for the diagnosis of OSA than a formal in-laboratory polysomnography (PSG). Many preliminary randomized trials of CPAP therapy for SDB in stroke patients have shown improvement in stroke scales, motor recovery, symptoms of sleepiness, and depression, but some randomized trials have shown no difference in outcome [249]. Despite inconsistencies, Davis et al. [263] made the following important conclusions regarding CPAP therapy for sleep apnea in stroke: Increased CPAP adherence improves stroke recovery, but adherence remains a problem because of advancing age, severity of stroke causing facial paralysis, dysphasia, and impaired cognition [249, 254, 263–267]. However, better selection, patient education, and early CPAP treatment during hospitalization or as soon as the patient can tolerate may improve increasing adherence and treatment benefit.
In addition to OSA, patients with stroke may have central sleep apnea (CSA), including central periodic breathing and CSB Figure (Fig. 41.16) [268–272]. CSA and CSB may persist in 6–29 % of stroke patients and may adversely affect the recovery [252]. Nopmaneejumrusler et al. [272] suggested that in patients with stroke, CSA and Cheyne–Stokes respirations are associated with hypocapnia and occult left ventricular systolic dysfunction but are not related to the location or type of stroke. Hermann et al. [271] found central periodic breathing during sleep in 3 of 31 patients with first-ever stroke in the absence of cardiopulmonary dysfunction. They assessed the patients using PSG, MRI of the brain, and echocardiography. They concluded that central periodic breathing during sleep may be present in strokes involving the autonomic (insular) and volitional (cingulate and thalamus) respiratory networks and that breathing improved in all patients during stroke recovery. Bonnin-Vilaplana et al. [268] described CSB in patients with first-ever lacunar stroke. In a recent retrospective analysis of adaptive servoventilation (ASV) treatment for persistent CSA in postacute ischemic stroke patients without concomitant congestive cardiac failure, Brill et al. [269] observed that ASV was well tolerated and clinically effective in these patients.


Fig. 41.16
Cheyne–Stokes breathing and central apnea: factors
Some studies have addressed circadian variations of stroke onset. In a study of 53 stroke patients, Kapen et al. [273] confirmed their previous reports of prevalence for the onset of stroke in the morning during a 6-hour period after awakening from sleep. This is similar to the peak incidence in the morning hours for myocardial infarction and sudden cardiac death. All of these conditions may be aggravated by a combination of circadian increase of corticosteroids and catecholamines, increased blood pressure and heart rate in the morning, and increased platelet “aggregability.” (Normal subjects exhibit increased platelet aggregability in the early morning [274]. In several other studies, the incidence of stroke was highest during sleep at night [275] or during early morning hours after awakening from nocturnal sleep [276, 277]. In order to investigate circadian variations in situations at stroke onset, Omama et al. [278] analyzed 12,957 cases of first-ever stroke onset diagnosed from the Iowa Stroke Registry between 1991 and 1996 by CT or MRI of the brain. They noted that patients who had cerebral infarctions showed a bimodal pattern with a higher peak in the morning and a lower peak in the afternoon, whereas intracerebral hemorrhage and subarachnoid hemorrhage patients had the same bimodal pattern but with a lower peak in the morning and a higher peak in the afternoon. The authors concluded that sleep tends to promote ischemic stroke and suppresses hemorrhagic stroke. Another study from Japan [279] found that intracerebral hemorrhage during the sleep period may be more detrimental compared with the intracerebral hemorrhage during awake periods, causing larger hematoma and higher mortality rates.
Brain Stem Vascular Lesions
Brain stem vascular lesions include infarction, hemorrhage, arterial compression, and localized brain stem ischemia. Sleep disturbances have been described in brain stem infarction by Markand and Dyken [280] and several other authors [19, 21, 281, 282]. Polysomnographic findings generally consisted of increased wakefulness after sleep onset and decreased REM and slow-wave sleep. Several reports of EEG or PSG studies to document sleep disturbances have been described in patients with locked-in syndrome, which is characterized by quadriplegia associated with de-efferentation and results from ventral pontine infarction. Patients are generally aware of their surroundings and are conscious. They cannot speak because of facial muscle paralysis but can respond by moving the eyes, whose control is spared. Sleep EEG recordings of locked-in syndrome patients have been reported by Feldman [283], Freemon et al. [284], Markand and Dyken [280], Cummings and Greenberg [285], Oxenberg et al. [286], and Nordgren et al. [287]. The EEG findings in these reports generally showed reduced or absent REM sleep and variable changes in NREM sleep, including reduction of slow-wave sleep and total sleep time. Oxenberg’s group [286], however, described only minor alterations in the initial recording in contrast to the more marked alterations noted in the other reports. The authors thought that the difference could be related to the extent of the lesion. Feldman [283] found in his patient reduced REM, stage 4 NREM, and total sleep time. Markand and Dyken [280] noted in five of seven locked-in syndrome patients the total absence of REM sleep and variable changes in NREM sleep. Cummings and Greenberg [285] described one patient who had reduced slow-wave sleep and another with reduced NREM sleep and no REM sleep. Autret et al. [288] also found a reduction of REM and NREM sleep in four patients after medial pontine tegmental stroke.
The term, Ondine’s curse, or the syndrome of primary failure of automatic respiration, was coined by Severinghaus and Mitchell [289] to describe three patients who experienced long periods of apnea even when awake but could breathe on command. They became apneic after surgery involving the brain stem and high cervical spinal cord and required artificial ventilation while asleep. When their consciousness was altered by nitrous oxide or thiopental, they became apneic. Carbon dioxide response to breathing showed low sensitivity. One patient died in apnea, and the two others improved in one week. The authors suggested that Ondine’s curse resulted from damage to the medullary carbon dioxide chemoreceptors. It is notable that the term Ondine’s curse was derived from the sea nymph in German mythology, whose curse rendered her unfaithful lover incapable of automatic respiratory function and caused his death. This eponymic syndrome generated considerable controversy and confusion [290]. The syndrome of Ondine’s curse is usually caused by bilateral lesions anywhere caudal to the fifth cranial nerve in the pons down to the upper cervical spinal cord in the ventrolateral region. Levin and Margolis [282] described a 52-year-old man with unilateral medullary infarction, however, who lost automatic respiratory control. At autopsy, the lesion was found to extend from the left lower pons through the left lateral medullary tegmentum to the upper cervical spinal cord and to involve the left paramedian PRF. Thus, in some patients, automatic respiratory control can reside unilaterally in the pontomedullary tegmentum.
A case of inverse Ondine’s curse syndrome in a patient with selective paralysis of voluntary respiration but preservation of automatic respiration was described by Munschauer et al. [291]. This was a 36-year-old man with sudden onset of quadriparesis and bulbar dysfunction in whom MRI demonstrated a well-demarcated lesion restricted to the ventral basilaris pontis. His hypercapnic ventilatory response and breathing during sleep were normal. Emotional stimuli producing laughter, crying, or anxiety appropriately modulated automatic respiration, but the patient could not voluntarily modify any respiratory parameters. The findings in this case suggested that descending limbic influences on automatic respiration are anatomically and functionally independent of the voluntary respiratory systems.
Bogousslavsky et al. [292] reported a clinical pathologic correlation in two patients who had central hypoventilation and unilateral infarct in the caudal brain stem. The authors suggested that unilateral involvement of the pontomedullary reticular formation and nucleus ambiguus is sufficient for generating a loss of automatic respiration, whereas associated lesion of the NTS may lead to more severe respiratory failure involving both automatic and voluntary responses [292].
Respiratory rate and pattern were studied by Lee et al. [19] by impedance pneumography in 14 patients with acute brain stem or cerebral infarction, and in a subsequent study, they reported on another 23 patients with acute brain stem infarction [21]. They found frequent abnormalities of respiratory pattern and rate in such patients, and these abnormalities became worse during sleep. The abnormal pattern included CSB and Cheyne–Stokes variant types of breathing, in addition to tachypnea and cluster breathing in some patients. In contrast to the observations of Plum et al. [11–13, 293] that such breathing patterns are associated with bilateral cerebral hemispheric and diencephalic lesions but rarely with lesions in the upper pons, Lee’s group [21] observed Cheyne–Stokes respirations in patients with extensive bilateral pontine lesions. They suggested that the size and the bilaterality of the lesions determined the types of respiratory pattern abnormalities.
Devereaux et al. [281] reported sleep apnea that required ventilatory support in two women who breathed normally while awake. Aged 36 and 59 years, they had bilateral infarctions limited to the lateral medullary tegmentum. In one of these patients, the carbon dioxide response was markedly depressed. Although the authors stated that acute automatic respiratory failure did not generally evolve into a chronic alveolar hypoventilation syndrome, their second patient continued to have sleep-induced apnea after many months.
Sleep apnea after bulbar stroke was also described by Askenasy and Goldhammer [294]. Their patient had a left-sided Wallenberg syndrome (lateral medullary syndrome), and 2 nights’ PSG recordings documented mostly obstructive or mixed apneas and hypopneas. This was a clinical diagnosis, and neuroimaging did not define the exact anatomy of the lesion. Their report, however, should direct attention to the possibility that unilateral brain stem lesions can cause sleep apnea syndrome. In such patients, it is important to diagnose and promptly treat ventilatory dysfunction during sleep.
Lassman and Mayer [295] described a 70-year-old woman with right lateral medullary infarction who developed recurrent episodes of life-threatening central hypoventilation requiring diaphragm pacing with a phrenic nerve pacemaker and nocturnal mechanical ventilation via a tracheostomy.
Diencephalic Stroke
Freund [296] should probably be credited with the first report of patients with hypersomnolence following paramedian thalamic strokes. Thalamic stroke may cause ipsilateral loss of sleep spindles [297], and bilateral paramedian thalamic infarcts may be associated with hypersomnia [298, 299]. Bassetti et al. [299] evaluated 12 patients with MRI-proven isolated paramedian thalamic stroke and hypersomnia. The patients were evenly divided between groups of severe and mild hypersomnia. Nocturnal PSG findings included increased stage 1 NREM sleep, reduced stage 2 NREM sleep, and a reduced number of sleep spindles. Bassetti et al. [299], however, found intact REM sleep as well as circadian, ultradian, and homeostatic sleep regulation in their patients. The authors concluded that hypersomnia after paramedian thalamic stroke is accompanied by deficient arousal during the day and insufficient spindling and slow-wave sleep production at night. Their observation supported the hypothesis of a dual role of the paramedian thalamus for the maintenance of sleep-wake regulation.
In contrast, Guilleminault et al. [300] reported three patients with pseudohypersomnia and presleep behavior with bilateral paramedian thalamic lesions. These authors used long-term monitoring with an infrared video camera and polygraphic study to document that their patients did not develop the normal NREM cycling during the day; rather, the EEG indicated a mixture of low-amplitude theta and alpha frequency waves during the day, with “sleep-like behavior.” The patients exhibited the behavioral aspects of sleep during the day, suggesting to Guilleminault et al. [300] that these subjects did not present hypersomnia but a “de-arousal” and were left in the transition between wakefulness and sleep. These authors [300] cited a report by Catsman-Berrevoets and von Harskamp [301] of a similar patient with compulsive presleep behavior and apathy due to bilateral thalamic stroke who responded to bromocriptine. There are a few other scattered cases reported of hypersomnolence following bilateral paramedian thalamic infarct [302–306]. Scammell et al. [307] described a narcolepsy-like syndrome with low CSF hypocretin-1 in a 23-year-old man after diencephalic stroke following the removal of a craniopharyngioma. Similar syndrome has been described by Castaigne et al. [298] after paramedian thalamic and midbrain infarcts.
Other Sleep-Wake Disorders in Stroke
Stroke may predispose to a number of other sleep disorders. Kleine–Levin syndrome can occur after multiple cerebral infarction [308]. Narcolepsy-cataplexy has been reported to follow cerebral hypoxia-ischemia [309].
Insomnia is commonly noted after cerebral infarction, but this may be partly due to the depression that typically follows stroke [310]. Bassetti and Hermann [264] reported an increased prevalence of sleep-wake disturbances in at least 20–40 % of stroke patients, mainly in the form of increased sleep need (hypersomnia), EDS, or insomnia. They listed several factors contributing to sleep-wake disorders in such patients, including depression, anxiety, SDB, complications resulting from stroke (e.g. nocturia, dysphagia, and urinary or respiratory infections), and medications. In another study by Palomaki et al. [311], the authors concluded that insomnia is a common complaint after ischemic stroke.
Total dream loss (Charcot–Wilbrand syndrome) after acute bilateral infarction of the deep occipital lobe as well as after parietal and deep frontal infarcts has been described by Bischof and Bassetti [312] and Solms [313].
Sleep architectural changes involving NREM and REM sleep have also been noted after cerebral hemispheric stroke [314–317].
Basal Ganglia Disorders
Sleep disturbances and sleep-related respiratory dysrhythmias are noted in many patients with basal ganglia disorders, but a systematic study to evaluate such dysfunction has not been undertaken in a large number of patients. A review of sleep and movement disorders is provided in Chap. 39.
Disorders of the Cerebellum
Cerebellar influence on the sleep-wakefulness mechanism has been clearly demonstrated in experimental animal studies by showing a mild decrement of NREM and an increment of REM sleep in cerebellectomized cats [326]. The role of the cerebellum in the respiratory control mechanism in sleep, however, is not known.
Olivopontocerebellar atrophy (OPCA) defines chronic progressive hereditary (usually dominant, occasionally recessive, rarely sporadic) cerebellar degeneration manifested by cerebellar-parkinsonian or parkinsonian-cerebellar syndrome and associated with atrophy of the pontine nuclei and cerebellar cortex and degenerative lesions of the olivopontocerebellar regions [327, 328]. There have been a few reports on sleep disturbances and sleep-related respiratory dysrhythmias in OPCA (for sporadic OPCA or multiple system atrophy [MSA], see further on).
Cerebellar role in sleep has also been demonstrated by showing changes in the sleep-wake cycle after lesions of the fastigial nucleus and middle cerebellar peduncle as well as EEG changes after electrical stimulation or suppression of cerebellar nuclei [329, 330]. Further evidence is shown by significantly increased activity in the cerebellum during slow-wave sleep following functional magnetic resonance imaging [331]. Brain stem neurons, which are known to be degenerated in OPCA [328], lie close to the hypnogenic [67] and respiratory neurons [82–85]. Thus, dysfunction of respiratory control, in parallel with the somatic structural dysfunction in OPCA, may be expected. The known morphologic changes of OPCA [328] are adequate to explain the sleep disturbances and sleep apnea in this condition. Several authors [332–336] described EEG sleep alterations in degenerative cerebellar atrophy. Reduced or absent REM sleep, reduced slow-wave sleep, and increased awakenings are the essential PSG findings. In several cases of OPCA, REM sleep without muscle atonia accompanied by the typical features of RBD has been described [336, 337]. Jouvet and Delorme [38] produced REM sleep without atonia in cats by bilateral pontine tegmental lesions. A similar lesion in OPCA may be also responsible for RBD in this condition. OPCA has also been associated with hyposomnia [338].
Sleep apnea has been described in several cases of OPCA [335, 338–340]. Patients with sporadic OPCA associated with prominent autonomic failure are now classified as having MSA or Shy–Drager syndrome (see Sect. “Sleep and Breathing Disorders in Autonomic Failure” later in this chapter). Chokroverty et al. [339] described five patients with OPCA and sleep apnea. PSG study showed repeated episodes of central, upper airway obstructive, and mixed apneas during sleep; the apneic episodes lasted from 10 to 62 s, and the apnea index was 30–55. Pure central apnea was noted in three patients, but all three types of apnea were seen in two, and most of the apneic episodes occurred during NREM sleep stage 2. Thus, these findings suggested central neuronal dysfunction in an area where respiratory and sleep-waking systems are closely interrelated, such as the NTS and the pontomedullary reticular formation. Salazar-Grueso and associates [335] described a 37-year-old man with a 19-year history of autosomal dominant OPCA and EDS whose PSG demonstrated episodes of mixed and central (predominantly central) sleep apnea and no sleep spindles or REM sleep. Trazodone treatment normalized the sleep architecture and reduced the apneic episodes.
Occasionally, sleep disturbances are associated with other types of cerebellar lesions, although systematic studies are lacking. Bergamasco et al. [341] made a polygraphic study of a 13-year-old girl with a diagnosis of dyssynergia cerebellaris myoclonica (Ramsay hunt syndrome). An all-night sleep study showed no REM sleep and increased slow-wave sleep. The EEG showed multiple spike-and-wave discharges accompanied by myoclonic generalized seizures. There are reports of sleep disturbances including RLS and RBD in spinocerebellar ataxia (SCA) type SCA1, SCA2, SCA3, as well as increased prevalence of SDB in Friedreich’s ataxia [329].
Brain Stem Tumor
Brain stem glioma with automatic respiratory failure was mentioned by Plum [13]. Ito et al. [342] described two children with brain stem gliomas and sleep apnea. Brain stem tumor may cause disorganization of the tonic and phasic events of REM sleep, as described in a patient whose pontine tumor caused a marked decrease in the atonia of REM sleep [343]. Lee et al. [344] reported a 74-year-old woman with recurrent acoustic neuroma at the cerebellopontine angle presenting as central alveolar hypoventilation. The patient had shallow breathing during sleep and had hypersomnolence during the daytime. Arterial blood gases showed increased Paco 2 and decreased partial pressure of arterial oxygen (Pao 2). Tumor resection eliminated hypersomnolence and respiratory failure. A patient seen by the senior author with a medullary tumor that caused severe hypoventilation during sleep required tracheostomy (unpublished observation). The central apneic episodes in the same patient became prolonged when the tracheostomy tube was occluded.
Other Brain Stem and Diencephalic Lesions
The metabolic and autonomic respiratory neurons and the lower brain stem hypnogenic neurons are located in the medulla. These neurons are influenced by the supramedullary respiration-controlling inputs and hypothalamic preoptic nuclei, as well as by the peripheral afferent inputs to the respiratory centers (see Chap. 9). Therefore, sleep and respiratory disturbances should be common manifestations of lesions in the brain stem, and many such cases have been described. Such disorders have included brain stem vascular lesions, tumors, traumatic lesions, multiple sclerosis (MS), bulbar poliomyelitis and post-polio syndrome, brain stem encephalitis, motor neuron disease affecting the bulbar nuclei, syringobulbia-syringomyelia, and Arnold–Chiari malformation [91]. In addition, several cases in which brain stem and diencephalic lesions caused narcolepsy-like syndrome have been described [307, 345–350]. All the characteristic features of narcolepsy are not seen in the cases. The causes have included infarction [307], trauma, tumors (including third ventricle tumor) [345], and arteriovenous malformation invading the third ventricle and affecting the hypothalamus [347], and some cases have been associated with MS [348–350].
Brain stem ischemic damage may also cause respiratory dysfunction. Beal et al. [351] described a 19-year-old man who had failure of automatic respiration and other signs of brain stem dysfunction after nearly drowning. He had sleep apneas, and PSG study confirmed the presence of CSAs. During wakefulness, his breathing was normal. Hypercapnic ventilatory response was markedly impaired, but hypoxic ventilatory response appeared to be normal. Autopsy findings 8 months later, after sudden death, documented marked bilateral neuron loss in the tractus solitarius, ambiguus, and retroambigualis nuclei. This most likely resulted from anoxia or ischemia.
Parenti et al. [352] described two patients aged 38 and 53 years with CSA who died during sleep. At autopsy, the authors described acute bilateral hypoxic lesions at the level of the solitary tract nuclei.
Traumatic Brain Injury and Sleep Dysfunction
Traumatic brain injury (TBI) is defined as “an alteration in brain function or other evidence of brain pathology caused by an external force” [353]. It includes concussion, contusion, laceration, hemorrhage, and cerebral edema. After a severe TBI, brain stem function is severely compromised and the patient becomes comatose. There have been many EEG studies in patients with coma, and some patients may demonstrate sleep patterns such as spindles and K complexes. Such patterns are designated as spindle coma [354]. It is often stated that the presence of EEG sleep patterns indicates a favorable prognosis [355], but this may not be necessarily true. On recovering from the coma during this stage of rehabilitation, many patients may have sleep-wake disturbances. However, there have been no adequate studies addressing the sleep-wake abnormalities in such patients. This is surprising, considering that one editorial labeled TBI a silent epidemic [356]. There is a dearth of studies addressing sleep-wake abnormalities after minor brain injuries that did not result in coma but caused a transient loss of consciousness. Many of these patients experience the so-called postconcussion syndrome, characterized by a variety of behavioral disturbances, headache, and sleep-wake abnormalities.
Traumatic brain injury is a public health challenge and a leading cause of death and disability according to the World Health Organization (WHO) [357, 358]. There is a high prevalence of sleep-wake (S-W) dysfunction in TBI including mild TBI adversely impacting recovery, rehabilitation, and outcome [359], but unfortunately S-W dysfunction is not routinely screened in such patients [360]. An important point to remember is that TBI may trigger post-traumatic stress disorder (PTSD) and depression as well as increased sensitivity to pain in such patients promoting and aggravating sleep dysfunction [359]. TBI may cause insomnia, sleep-disordered breathing (SDB), hypersomnolence not due to SDB, circadian rhythm sleep disorders (CRSD), parasomnias, and sleep-related movement disorders (Fig. 41.17). In the past 15 years, there has been an explosion of studies using both self-report and objective methods to characterize sleep dysfunction in TBI [358–381].


Fig. 41.17
Sleep dysfunction in traumatic brain injury TBI)
In a meta-analysis involving 21 studies including 1706 subjects with mild, moderate, and severe TBI, Mathias and Alvaro [359] reported that overall, 50 % of subjects had some form of sleep disturbance and 25–29 % had a specific sleep disorder (insomnia [29 %], hypersomnia [28 %], obstructive sleep apnea [25 %], PLMS [19 %], and post-traumatic narcolepsy [4 %]). These rates are significantly higher than those seen in the general population. The authors suggested routine screening for sleep dysfunction in TBI patients for optimal treatment and favorable outcome and recovery. Similar suggestion was made by Mollayeva et al. [360] in a later review. In a prospective longitudinal study in 51 consecutive TBI patients, Kempf et al. [371] showed that three years after TBI, two out of three patients had a significant S-W disturbance, particularly post-traumatic hypersomnia, and only 10 % of patients had insomnia.
Verma et al. [365] studied 60 TBI patients (40 % mild, 60 % moderate-to-severe; three months to two years post-trauma) from a sleep clinic and observed the following S-W disorders: sleep apnea (30 %), parasomnias (25 %) including REM sleep behavior disorder (RBD) or increased EMG tone during REM sleep (13 %), sleepwalking (8 %), nightmares (7 %), sleep paralysis (5 %), nocturnal enuresis (5 %), nocturnal eating disorder (3 %), and post-traumatic narcolepsy (3.3 %).
There are several studies investigating the frequency of sleep disorders in TBI patients with hypersomnia and insomnia. Baumann et al. [381] prospectively assessed CSF hypocretin-1 levels in 44 consecutive patients with acute TBI. They found abnormally lower hypocretin-1 levels in 95 % of patients with moderate-to-severe TBI compared with controls. Later on, this group of authors [382] enrolled 96 consecutive patients within the first 4 days after TBI, attempting to delineate the frequency and clinical characteristics of post-traumatic sleep-wake disorders. Six months later, they studied 65 of these patients using questionnaire, CT scan of the brain, CSF hypocretin-1 levels, overnight PSG, and MSLT and actigraphy. They found new-onset sleep-wake disorders following TBI in 72 % of these patients, objective EDS in the MSLT in 25 %, post-traumatic hypersomnia (increased sleep need of 2 or more hours within 24 h compared to the pre-TBI period) in 22 %, and insomnia in 5 %. They found low CSF hypocretin-1 levels in 4 of 21 patients 6 months after TBI compared to 25 of 27 patients in the first few days after TBI. The patients with post-traumatic sleep-wake disorders also had impaired quality of life. The authors suggested that sleep-wake disturbances are common and involvement of the hypocretin system is possible in the pathophysiology of post-traumatic hypersomnia.
Castriotta et al. [383] evaluated 87 adult patients at least 3 months after TBI using overnight PSG, MSLT, ESS, and neuropsychologic testing. They found a high prevalence of sleep disorders (46 %) and of EDS (25 %) in subjects with TBI. Sleep dysfunction in these patients included a high prevalence of OSA (23 %), post-traumatic hypersomnia (11 %), post-traumatic narcolepsy (7 %), and PLMS (7 %). Ouellet and coinvestigators [384] evaluated 452 subjects aged 16 years and older with minor-to-severe TBI utilizing a questionnaire related to quality of sleep. They found overall insomnia symptoms in 50.2 % and diagnostic criteria for an insomnia syndrome in 29.4 %. Risk factors for insomnia included mild TBI and high levels of fatigue, depression, and pain. Ouellete and Morin [385] studied 14 patients with mild-to-severe TBI compared to 14 healthy good sleepers using a sleep diary and 2 nights of overnight PSG. The authors found a higher proportion of stage 1 sleep in the TBI participants than in controls, but the percentages of stage 2, slow-wave, and REM sleep did not differ between the two groups. TBI patients, however, had more awakenings lasting longer than 5 min and a shorter REM sleep latency. The authors concluded that these results were similar to those found in patients with either primary insomnia or insomnia comorbid with depression.
Schreiber et al. [386] observed alterations in both timing and sleep architecture in 26 adult patients with a past history of minor head trauma (no structural brain imaging findings). In contrast, Gosselin and investigators [387] observed only subjective complaints but no objective sleep architectural abnormalities in 10 athletes with a history of sport-related concussion within the past year compared with 11 nonconcussed athletes. In a subsequent investigation, this group [388] confirmed their previous observations of a lack of abnormal sleep macroarchitecture in mild TBI patients but observed an increase of EEG beta power which could be attributed to brain injury or other factors such as anxiety or pain.
Post-traumatic hypersomnia has been listed in the third edition of the International Classification of Sleep Disorders (ICSD-3) under the heading “Hypersomnia due to a medical disorder” [389]. Guilleminault et al. [390] evaluated 20 patients with post-traumatic hypersomnia using PSG and the MSLT. The causes were multiple, including cases secondary to sleep apnea syndrome. TBI may cause central and upper airway obstructive sleep apnea by inflicting functional or structural alterations of the brain stem respiratory control system. It is important to remember, however, that many patients may have sleep apnea syndrome before sustaining TBI.
EDS in adults with TBI was also reported prospectively in case series of subjects by Castriotta and Lai [391]. These investigators found a high prevalence of sleep apnea-hypopnea syndrome, PLMS, and post-traumatic hypersomnia as well as post-traumatic narcolepsy. Post-traumatic narcolepsy in mild-to-moderate head injury in nine patients was also reported by Lankford et al. [392] utilizing overnight PSG and MSLT.
Hypersomnia or increased sleep need per 24 hours is noted in TBI in association with EDS, by the group led by Baumann [364] who suggested the term pleiosomnia for the increased need for sleep following TBI indicating an increased pressure for sleep. In a recent review of 12 cases of recurrent hypersomnia following TBI, Billiard and Podesta [370] attributed two directly to TBI. Collen et al. [374] retrospectively studied 116 soldiers with combat-related TBI and reported EDS in 85.2 % of the participants. These combat veterans also had a high rate (90.5 %) of comorbid psychiatric disorder (e.g., PTSD, anxiety, and depression).
Okawa et al. [393] described disturbance of circadian rhythms in severely brain-damaged patients. Patten and Lauderdale [394] reported a case of delayed sleep phase syndrome in a 13-year-old boy after a minor head injury sustained in a motorcycle accident (5 min’ loss of consciousness followed by headache and drowsiness without other objective neurologic findings). Quinto et al. [395] reported a case of a delayed sleep phase syndrome (DSPS) in a 48-year-old man after TBI. There is another report of post-traumatic DSPS in a 15-year-old girl treated successfully with 5 mg melatonin [396]. In a more recent study, Ayalon et al. [397], using actigraphy, salivary melatonin, oral temperature measurement, and PSG, evaluated 42 patients with mild TBI and found circadian rhythm sleep disorders (DSPS in eight, irregular sleep-wake pattern in seven) in 36 % (15 patients).
The pathophysiology of post-traumatic S-W dysfunction remains unclear but most likely results from a complex interaction of anatomic, physiologic, environmental, and psychologic (e.g., anxiety, depression, and maladaptive habits) factors, as well as neurotransmitters and neuromodulators including hypocretin levels and hormonal changes, circadian rhythms, age, pain, and genetic predisposition.
In summary, TBI of any severity (mild, moderate, severe) may cause a variety of sleep-wake disorders with a much higher prevalence than in general population, impacting on quality of life and final outcome of rehabilitative and other therapies (schematically shown in Fig. 41.17).
Demyelinating Lesion in the Brain Stem
Sleep disturbances are very common in MS and may be seen in over 50 % of patients [398–454]. Surprisingly, there is a lack of adequate evaluation and characterization of such dysfunction in a systematic manner using subjective and objective measures in MS patients. Most MS patients suffer from an unexplained fatigue (see Chap. 42). An important reason for such fatigue may be an unsuspected sleep disorder, such as SDB or insufficient nocturnal sleep. Sleep disturbances in MS may include insomnia, hypersomnia, SDB, narcolepsy, restless legs syndrome (RLS)-PLMS, and RBD [398–454]. We do not know whether such sleep disturbances have an adverse impact on the natural history of MS (Fig. 41.18). Also, we have insufficient knowledge about the effects of treatment of MS with immunomodulating therapies on sleep-wake disturbances (see further on). It is not known whether sleep-wake disorder is related to the severity of the illness as there have not been adequate studies in a large number of MS patients complaining of sleep-wake disorder correlating with Kurtzke’s expanded disability scale score.


Fig. 41.18
Types of sleep dysfunction and factors: multiple sclerosis
Sleep-Disordered Breathing in MS
In individuals with MS, a demyelinating plaque may involve the hypnogenic and respiratory neurons in the brain stem, giving rise to sleep disturbance and SRBDs. A few such cases have been described in MS patients. An unusual patient, a 38-year-old man who had a clinical diagnosis of acute demyelinating lesion in the cervicomedullary junction, was described by Newsom Davis [398]. The patient had an autonomous breathing pattern, but he could neither take a voluntary breath nor stop breathing, thus illustrating the apparent independence of the mechanisms controlling metabolic and behavioral respiratory control systems. A patient reported by Rizvi et al. [399], whose brain stem dysfunction was consistent with MS, became apneic when asleep but was able to breathe when awake. His hypercapnic and hypoxic ventilatory responses were normal. Boor and associates [400] described a 40-year-old patient with paralysis of automatic respiration. During relaxation, the patient had recurrent apnea, but the breathing was stable when the patient was alert. The discovery at postmortem examination of a large, demyelinating lesion in the central medulla involving the medullary respiratory neurons explained the respiratory failure.
There are other reports of SDB [401–407] in MS, including failure of automatic respiration (Ondine’s curse) [408] with sudden respiratory arrest and nocturnal death, CSA, hypoventilation, and paroxysmal hyperventilation. It is important to consider such a possibility when patients complain of EDS, fatigue, and disturbed sleep associated with snoring. Figures 41.19 and 41.20 show a sample of the hypnogram and overnight PSG recording from a 51-year-old woman with a history of MS showing frequent obstructive, mixed, and central apneas and hypopneas during both NREM and REM sleep associated with mild-to-moderate oxygen desaturation and frequent arousals, as well as REM sleep dysregulation with longer REM sleep in the early part of the night.



Fig. 41.19
A case of SDB in a 51-year-old woman with a history of multiple sclerosis diagnosed 7 years ago. Her sleep difficulties started approximately 3 years ago and are described as frequent night awakenings, sleepwalking, and brief episodes consisting of sudden sleepiness or impairment of consciousness resulting in falls and multiple fractures but never accompanied by jerky movements of the limbs, tongue biting, or incontinence, with spontaneous recovery in 5–10 min without residual confusion. These episodes occur during the early morning hours as well as during the day. Her neurologic examination is significant for the presence of decreased visual acuity and impaired saccades bilaterally, horizontal nystagmus on looking to the left, intention tremor (left more than right) on finger-to-nose testing, mild ataxia in the lower extremities on heel-to-shin testing, tandem ataxia, and impaired joint and position sense in the toes bilaterally. She was clinically evaluated with a differential diagnosis of SDB related to multiple sclerosis, narcolepsy-cataplexy secondary to multiple sclerosis, and sleepwalking. Unusual nocturnal seizures remained unlikely given the clinical features, the several negative electroencephalograms, and the negative long-term epilepsy monitoring. This hypnogram is significant for REM sleep distribution abnormality (the longest REM in the early part of the night); frequent, obstructive, mixed, and central apneas and hypopneas both during NREM and during REM sleep; mild-to-moderate O2 desaturation; and frequent arousals. Sleep-related respiratory dysrhythmias due to brain stem involvement are a common finding in multiple sclerosis patients. Reproduced with the permission from Chokroverty et al. [455]

Fig. 41.20
A 120-s excerpt from an overnight polysomnographic recording (same patient as in Fig. 41.19) showing repeated central apneas with O2 desaturation. An increase in muscle tone is noted on chin (chin EMG) and tibialis anterior (Tib.) electromyography (EMG) channels following some central events. Electroencephalogram (EEG): top 10 channels. Lt. left; Rt. right; EOG electrooculograms; P Flow, peak flow; oronasal thermistor; chest and abdomen effort channels; snore monitor; EKG electrocardiography; SaO2, oxygen saturation by finger oximetry. Reproduced with the permission from Chokroverty et al. [455]
Insomnia in MS
In addition to sleep-related breathing abnormalities, other sleep difficulties have been commonly reported in MS patients, including insomnia and EDS [401, 402, 431–435, 440, 441]. Insomnia is the most common sleep problem in MS with an overall prevalence of more than 40 %. Tachibana et al. [431] evaluated 28 consecutive patients with MS, 15 of whom had sleep problems that included difficulty initiating sleep, frequent awakenings, difficulty maintaining sleep, habitual snoring, and nocturia. All-night oximetric study showed sleep-related O2 desaturation in three patients, two of whom had sleep apnea on PSG investigations. The authors concluded that sleep disturbance in MS is common but poorly recognized. Ferini-Strambi et al. [401] performed PSG studies in 25 MS patients and compared the results with 25 age- and sex-matched controls. They found reduced sleep efficiency with increased awakenings during sleep and an excess of PLMS in patients as compared with the controls. The cross-sectional study of Veauthier et al. [404] quoted 25 % prevalence of insomnia in MS patients, but most other studies in MS did not consider standardized diagnostic criteria confounding a methodological limitation. Sleep disturbance causing repeated arousals and sleep fragmentation is thought to be a contributing factor for MS-related fatigue [402, 436] (see also Chap. 42). An important cause of insomnia in MS is comorbid depression, which has a high prevalence (up to 50 %); it is important to recognize depression as treatment may improve sleep dysfunction and quality of life [417–419, 428, 430, 443–446, 454].
RLS-PLMS has a high prevalence in MS patients and in the general population [420, 435]. RLS-PLMS is an important cause of insomnia in these patients. Manconi et al. [435] studied 156 MS patients using a structured questionnaire and assessing the ESS; about one-third of their subjects satisfied the criteria for RLS. These authors noted that the primary progressive form of MS was more representative of the RLS group, which showed a higher ESS score than those without RLS. In a later multicenter Italian REMS study, Manconi et al. [447] reported that the prevalence of RLS was 19 % in MS and 4.2 % in controls. They identified the following risk factors: older age, longer duration, primary progressive MS form, higher disability, and the presence of leg jerks at sleep onset.
A recent meta-analysis by Schurks and Bussfeld [420] covering 24 studies estimated fourfold higher risk of RLS in MS patients than in those without MS. Manconi and collaborators [421] based on diffusion tensor MRI study suggested that cervical cord damage was a significant risk factor for RLS in MS which agrees with previous suggestion by Hartmann et al. [422]. A disconnection between brain stem and spinal cord due to interruption of ascending or descending pathways from the cervical spinal cord may be provided as an explanation for RLS in these patients [409].
Fatigue, Sleep Dysfunction, and MS
Most patients with MS suffer from an unexplained fatigue (see also Chap. 42). In the studies by Attarian et al. [436], Kaynak et al. [402], and others [415], there is a significant correlation between fatigue and disrupted sleep with sleep fragmentation, alteration of sleep macrostructure and microstructure, poor quality of life, and depression.
Other Sleep Disturbances in MS
Hypersomnolence with low CSF hypocretin-1 levels in primary and secondary narcolepsy [423–425, 438, 449, 450–453] and RBD [439] have also been observed in some MS patients. Plazzi et al. [439] described a 25-year-old woman with MS who presented with RBD as an initial presentation of MS, and this subsequently resolved after treatment with adrenocorticotrophic hormone. No MRI documentation, however, of brain lesions was provided. More recently, Tippmann-Peikert et al. [426] reported a 51-year-old woman with acute MS and RBD showing a large MS plaque (MRI T2 signal) in the dorsal pons similar to the lesion site causing RBD-like behavior in cats. One study [427] estimated prevalence of RBD in MS as 1.4 %.
Medications Causing Sleep Disturbance in MS
Treatment of MS with immunomodulating therapies such as interferon and methylprednisolone may cause sleep disturbances in the form of hypersomnolence, increasing fatigue, insomnia, and depression [454, 456]. The newer medications such as glatiramer acetate (Copaxone) or mitoxantrone (Novantrone) have not been found to cause sleep disturbance in MS, but adequate studies have not been undertaken. Insomnia, however, has been reported with laquinimod treatment in MS patients [457]. No specific information is available for natalizumab and fingolimod [409]. There is a report [458] showing that compared with oral baclofen, intrathecal infusion to treat severe spasticity in MS patients improved sleep continuity without affecting respiratory function.
Quality of Life and Sleep Dysfunction in MS Patients
Merlino et al. [440] studied 120 MS patients to assess the prevalence of sleep dysfunction in MS and to evaluate various factors affecting sleep quality and quality-of-life indicators. These authors found poor sleep in 47.5 % of the patients and concluded that poor sleep is an independent predictor of impaired quality of life, directing our attention to assessment and treatment of sleep dysfunction in MS patients to improve the quality of life. They also confirmed significantly higher mean Kurtzke MS disability scores among poor sleepers than among good sleepers, supporting an earlier report by Lobentanz et al. [446] but contradicting other reports [431, 432, 439] showing no correlation with these scores.
Mechanisms of Sleep Dysfunction in MS
Sleep-wake disturbances in MS may result from a variety of factors (see Fig. 41.18) as listed below: discomfort from immobility, spasticity and pain, nocturia, abnormal motor activities (e.g., RLS-PLMS, muscle spasms, and parasomnias), anxiety, depression, sleep-disordered breathing, anatomic locations of MS plaques (e.g., location of lesions affecting sleep-wake regulatory regions in brain stem, hypothalamus, and other brain areas), immunologic and cytokine abnormalities, and genetic predisposition (in some cases).
Bulbar Poliomyelitis and Post-polio Syndrome
In the acute and convalescent stages of poliomyelitis, respiratory disturbances commonly get worse during sleep. Some patients are left with the sequelae of respiratory dysrhythmia, particularly sleep-related apnea or hypoventilation requiring ventilatory support, especially at night. Another group of patients decades later develop symptoms that constitute post-polio syndrome. Sleep disturbances and sleep apnea or hypoventilation are also noted in post-polio syndrome. Medullary respiratory and hypnogenic neurons are involved directly in the poliovirus infection, and this explains the patients’ symptoms.
Bulbar Poliomyelitis
Hypoventilation syndrome in bulbar poliomyelitis was first documented quantitatively by Sarnoff et al. [459]. They described four patients who could breathe voluntarily on command but hypoventilated during periods of sleep and quiescence. The authors described irregular rate and rhythm of respiration, incoordination of the muscles of respiration, and hypoventilation resulting from decreased sensitivity of the respiratory center to Paco 2 as a result of direct involvement of the respiratory center by the poliomyelitis virus. Two of their patients benefited from electrophrenic respiration.
An extensive report on the clinical and physiologic findings in 20 of 250 poliomyelitis patients with central respiratory disturbances was given by Plum and Swanson [16]. These patients’ respiratory disturbances could not be explained by involvement of the spinal motor neurons or airway obstruction. In acute bulbar poliomyelitis, the disordered breathing progressed through three successive stages. Stage I was characterized by disorder of respiratory rhythm during sleep, when breathing became irregular in rate and depth with periods of apnea ranging from 4 to 12 s. During stage II, normal breathing required increasing effort and concentration, and strong auditory or painful stimuli were necessary to maintain respiratory rhythmicity. At this stage, the patients had impaired chemosensitivity of the central respiratory centers as evidenced by a reduction in ventilation and carbon dioxide retention after O2 inhalation. Sleep exacerbated the breathing difficulty, and there were longer periods of apnea. The respiratory homeostasis was lost entirely in stage III, and there was no ventilatory responsiveness to reflex, chemical, or other neuronal stimuli. The respiratory pattern was chaotic, with varying periods of apnea. The patients required ventilatory support to maintain respiratory homeostasis. Severe inflammatory changes and small areas of necrosis in the ventrolateral reticular formation of the medulla were noted in two patients on neuropathologic examination.
The breathing abnormalities in this series rarely lasted more than 2 weeks, but two patients had sleep-related irregular respiration that persisted many months after acute poliomyelitis. These two patients also demonstrated impaired hypercapnic ventilatory response and hypoventilation during administration of 100 % O2. The physiologic abnormalities suggested severe and permanent dysfunction of the medullary respiratory neurons. In several convalescent spinal poliomyelitis patients, the authors also observed subnormal ventilatory response to carbon dioxide with reduction of maximum breathing capacity or vital capacity to less than 50 % of predicted normal values. These findings implied that peripheral mechanisms that cause restriction of chest movements may also contribute to impaired ventilatory response to carbon dioxide.
Post-polio Syndrome
Post-polio syndrome is manifested clinically by increasing weakness or wasting of the previously affected muscles and by involvement of previously unaffected regions of the body, fatigue, aches and pains, and sometimes symptoms secondary to sleep-related hypoventilation, such as EDS and tiredness [460, 461]. The exact mechanism of post-polio syndrome is not known. Some of the symptoms (e.g., EDS and fatigue) could result from sleep-related hypoventilation or apnea and sleep disturbances [462]. Thus, it is important to be aware of sleep apnea in such patients. This syndrome has been described in patients who had poliomyelitis decades earlier. Guilleminault and Motta [463] reported on five such men who had a history of bulbar poliomyelitis 16 years earlier. All had EDS, and PSG study documented numerous episodes of apneas, which were predominantly central but also mixed and upper airway obstructive types associated with O2 desaturation. Their longest apneas were seen during REM sleep. It is important to know that these patients resemble those with primary sleep apnea syndrome. Presumably, the lesions in these cases involved the medullary respiratory neurons, and thus, central lesions were responsible for all three types of apneas. The patients’ symptoms improved and daytime somnolence decreased after ventilatory assistance at night.
Steljes et al. [462] performed PSG examinations on 13 post-polio patients, 5 of whom used rocking beds for ventilatory assistance and 8 of whom had no ventilatory assistance. Patients who required ventilatory assistance demonstrated severe sleep disturbances with decreased total sleep time, reduced sleep efficiency, and decreased percentages of stage 2 NREM sleep, slow-wave sleep, and REM sleep, but increased awakenings and percentage of stage 1 NREM sleep. Respiratory abnormalities in these patients consisted of hypoventilation, apneas, and hypopneas associated with significant O2 desaturation. These patients did not respond to CPAP treatment with the rocking bed, but they showed improvement in sleep structure and respiratory function after mechanical ventilation via nasal mask. Five of the eight patients who required no ventilatory assistance also showed impairment of sleep architecture similar to the other group, but the findings were less severe. All but one patient from the second group had obstructive or mixed apneas, which were treated successfully with nasal CPAP. One patient with mixed apnea and marked hypoventilation improved after treatment with nasal ventilation by mask.
Polysomnographic and pulmonary function studies by Bye et al. [464] and Ellis et al. [465] documented respiratory failure and sleep hypoxemia, particularly during REM sleep, in patients with post-polio respiratory muscle weakness. Sleep studies by Ellis’ group [465] under controlled conditions without respiratory support showed repeated arousals with disruption and fragmentation of the REM-NREM cycle. In a retrospective review of medical records from 108 consecutive patients with post-polio syndrome and sleep disturbances encountered during an 11-year period at the Mayo Clinic, Hsu and Staats [466] reported PSG findings from 35 patients fulfilling the inclusion criteria. All patients had hypersomnolence as the most common presenting symptom, and the authors identified three patterns of sleep disturbances: OSA, hypoventilation, and a combination of both. They concluded that SDB is a late sequela of poliomyelitis. Dean et al. [467] from the National Institutes of Health reported the PSG findings in 10 patients with clinical signs of post-polio syndrome. They noted disruption of sleep architecture due to sleep apnea (both central and obstructive), which was more frequent in patients with bulbar involvement who had more central than obstructive apneas. Bruno [468] reported abnormal movements during sleep studies (e.g., random myoclonus, brief ballistic and slow grasping movements, and PLMS) in seven poliomyelitis survivors. A physiologic study to understand the sequence of events during REM sleep in 13 patients with post-polio syndrome by Siegel et al. [469] from the National Institutes of Health measured latencies to the onset of the first occurrence of muscle tone reduction, the first sawtooth waves, and the first REMs in 13 patients with post-polio syndrome. The latencies for the entire group were longer than those of the normal volunteers, and the latencies for the bulbar group were significantly longer than those for the nonbulbar group of post-polio patients. The authors concluded that prolongation of these latencies may be due to prolonged recruitment time for neurons in the pontine tegmentum as a result of damage from the poliomyelitis virus in the past.
In a more recent report, Marin et al. [470] observed 10 fulfilling the diagnostic criteria for RLS/WED (2 men, 8 women; mean age of 42.5 years) among 35 post-polio patients in the outpatient clinics. They noted a concomitant occurrence of both RLS and post-polio patients suggesting a possible common underlying mechanism for both conditions. This is a small sample without controls, and epidemiologic studies including larger samples are needed to confirm this relationship. Silva et al. [471] in a brief report described some nonspecific sleep architectural changes, increased PLMS, and apnea-hypopnea index in overnight polysomnographic studies obtained from 60 randomly selected post-polio patients. The authors concluded these abnormalities reflected a dysfunction of the surviving motor neurons in the brain stem. There have been no new advances in the management of post-polio patients [472].
Syringobulbia-Syringomyelia
Some patients with syringobulbia-myelia may have alveolar hypoventilation and sleep-related apneas or irregular breathing and stridor. Haponik et al. [473] described such a case. The patient was a 35-year-old woman whose polygraphic examination showed 370 upper airway obstructive apneas lasting 10–170 s associated with hypoxemia during 7 h of NREM stages 1 and 2 sleep. The patient died 9 months after the onset of the illness, and neuropathologic examination disclosed a syrinx that extended from the lower third of the medulla to the upper thoracic spinal cord. Nogues et al. [474] studied 30 patients with syringomyelia, including 17 with syringobulbia, using overnight PSG and pulmonary function tests that also included hypercapnic ventilatory response; they found SDB (central, mixed, and obstructive apneas) in 1 of 13 patients with syringomyelia and 13 of 17 patients with syringobulbia. Impaired hypercapnic ventilatory response was noted in patients with syringobulbia. They found that symptoms of dysphagia and dysphonia rather than the size of the cavity on MRI or muscle weakness were predictive of SDB. These authors [475] also reported PLMS during NREM stages 1 and 2 in 16 of 26 patients with syringomyelia and periodic limb movements in wakefulness in three of these patients. The EMG latency delay between the upper and lower limb muscles suggested conduction along slowly conducting propriospinal pathways, indicating spinal cord hyperexcitability similar to the propriospinal propagation of PLMS in some RLS patients [476]. An occasional report [477] of postural tachycardia syndrome (see later) in syringomyelia and cardiovascular autonomic [478] impairment associated with sleep apnea in syringomyelia and syringobulbia suggest involvement of brain stem and spinal autonomic neurons. In a recent review published in 2012, Massimi et al. [479] stated that in the last three decades, only 41 cases of abrupt onset of syringomyelia associated with Chiari malformation type 1, respiratory failure (29 %), and cardiac autonomic dysfunction have been reported.
Sleep Dysfunction and Encephalitis
Cohn and Kuida [480] and White et al. [481] described alveolar hypoventilation, CSA, EDS, and subnormal hypercapnic ventilatory response after western equine encephalitis. The respiratory center was thought to have been damaged by the virus. Iranzo et al. [482] reported six patients with nonparaneoplastic limbic encephalitis associated with antibodies to voltage-gated potassium channels. Five of these patients had RBD associated with the onset of limbic encephalitis and in three of these immunosuppression resulted in the resolution of RBD in parallel with remission of the limbic syndrome. The authors suggested that RBD may be seen in the setting of voltage-gated potassium channel antibody-associated limbic encephalitis, which may be related to an autoimmune-mediated mechanism.
Sleep dysfunction, including total insomnia, NREM and REM parasomnia, has been described in many other types of autoimmune (antibody-mediated) encephalopathies [483–485] (e.g., anti-NMDA-receptor encephalitis and several limbic encephalitis subtypes). Distinctive clinical features including sleep disorders along with neuroimaging and electrophysiologic findings may indicate specific antibody-mediated encephalitis. A novel encephalopathy (a tauopathy) associated with cell surface autoimmunity (IgLON5) causing a novel NREM and REM parasomnia (RBD) with OSA has been recently described [486].
Arnold–Chiari Malformation
Arnold–Chiari malformation, particularly types I and II, may cause SDB, predominately CSA but also upper airway OSA, and central hypoventilation, including sudden respiratory arrest during sleep or postoperatively [487–489]. A repeat PSG in 6 of 12 patients out of 16 consecutive patients with Arnold Chiari malformation type I showed a decrease in the central apnea index following decompression surgery [490]. In a study by Sergio et al. [491] reporting 103 patients with Arnold–Chiari malformation (36 with type I and 67 with type II), a video-PSG study showed RBD in 23 and SDB in 65 (predominantly with CSA syndrome in 61 of the 65 patients). More recently, PSG documented a high prevalence of sleep apnea (both central and obstructive) in 16 children with Chiari type II [492] and eight out of 22 children with Chiari type I [493] malformations who showed significant improvement of central apneas after surgical decompression. Figures 41.21 and 41.22 show a hypnogram and a 120-second excerpt from a PSG recording from a 52-year-old man with Arnold–Chiari malformation type I. The hypnogram findings suggest REM sleep-related hypoventilation, and the PSG tracing shows obstructive apneas and hypopneas.



Fig. 41.21
A case of Arnold–Chiari malformation and SDB in a 52-year-old man with a history of tiredness and excessive daytime sleepiness for many years but no cataplexy, sleep paralysis, or hypnagogic hallucinations. At the age of 27 years, he complained of gait problems, and magnetic resonance imaging examination revealed Arnold–Chiari malformation type 1. His neurologic examination is significant for the presence of a coarse horizontal nystagmus, minimal right lower facial weakness, minimal right wrist extensor muscle weakness, minimal-to-mild ataxia in upper and lower extremities on coordination testing, and the presence of ataxia on tandem gait. This hypnogram shows a few periods of apneas and hypopneas accompanied by mild-to-moderate oxygen desaturation and arousals limited exclusively to a single REM sleep period recording during the night. A supine posture is maintained throughout the polysomnographic recording. These findings are suggestive of REM sleep-related hypoventilation. Central sleep apnea, obstructive sleep apnea, and hypoventilation have all been described in patients with Arnold–Chiari malformation, likely from brain stem involvement. Reproduced with the permission from Chokroverty et al. [455]

Fig. 41.22
A 120-s polysomnographic excerpt from REM sleep (same patient as in Fig. 41.21) showing one obstructive apnea followed by two sequential hypopneas. Oxygen desaturation of 82 %, likely from a prior respiratory event, is recorded at the onset of the epoch. The first two events are followed by an arousal response, and the epoch does not include the complete recovery phase of the third event. Phasic eye movements of REM sleep are noted on the electrooculogram (EOG) channels in the early part of the epoch. Phasic muscle twitches of REM sleep are noted on both tibialis anterior electromyography (Tib. EMG) channels. Chin EMG channel shows electrocardiography artifact. Electroencephalogram (EEG): top 10 channels. Lt. left; Rt. right; P Flow peak flow; oronasal thermistor; chest and abdomen effort channels; snore monitor; EKG, electrocardiography; SaO2, oxygen saturation by finger oximetry. Reproduced with permission from Chokroverty et al. [455]
Diseases of the Spinal Cord
In spinal cord disorders, sleep disturbances occur as a result of sleep-related respiratory dysrhythmias causing sleep apneas, hypopneas, or hypoventilation associated with hypoxemia and repeated arousals. The voluntary or behavioral respiratory control system descends via the corticospinal tracts, and the metabolic or automatic respiratory control system descends via the reticulospinal tracts; the two systems are integrated in the spinal cord (see also Chap. 11). The behavioral system is located in the dorsolateral quadrant of the cervical spinal cord [13, 14] and the automatic respiratory system in the ventrolateral quadrant. These two systems control the final common respiratory pathways of the spinal respiratory motor neurons, which send impulses along the phrenic and intercostal nerves to the main respiratory muscles. The anterior horn cells in the third, fourth, and fifth cervical spinal cord segments give rise to phrenic nerves, and the intercostal nerves originate from the ventral rami from the anterior horn cells in the thoracic spinal cord. It is known that transection of either the dorsolateral or the ventrolateral quadrant of the spinal cord may independently affect the voluntary and the automatic respiratory control systems [79]. Most reports, however, refer to transection of the ventrolateral tracts giving rise to dysfunction of the metabolic respiratory control system. Direct involvement of the lower motor respiratory pathways, either in the anterior horn or in the phrenic and intercostal nerves, may also give rise to respiratory dysfunction. Three patterns of respiratory dysfunction have been summarized by Krieger and Rosomoff [494]: (1) efferent motor impairment (e.g., phrenic nerve paralysis causing diaphragmatic weakness) associated with reduced vital capacity, (2) impaired hypercapnic ventilatory response without significant chest wall or diaphragmatic weakness and with normal vital capacity, and (3) a mixture of these two abnormalities. The lesions that cause such dysfunction in the spinal cord may include spinal surgery, spinal trauma, amyotrophic lateral sclerosis (ALS), syringomyelia, cervical spinal cord tumor, and cervical myelitis (demyelinating or nonspecific myelitis).
Spinal Surgery
Several cases of sleep apnea have been described after spinal surgery [494–496]. Krieger and Rosomoff [495] also described sleep apnea after high cervical cordotomy. These authors observed respiratory dysfunction within 24–48 h of bilateral percutaneous cervical cordotomy in 10 patients [494]. Although the authors concluded that the ascending reticular fibers in the ventrolateral segment of the spinal cord that relay afferent impulses to the medullary respiratory center had been damaged in their patients, it is most likely that selective damage to the descending automatic respiratory control fibers in the ventrolateral quadrant of the spinal cord was the lesion responsible. In two other patients, Krieger and Rosomoff [495] described sleep apnea that required assisted ventilation at night for several days after anterior spinal surgery at C3-4 interspace. Lahuerta et al. [496] performed high cervical cord percutaneous cordotomy for pain management in 12 patients, all of whom died during sleep postoperatively, presumably from respiratory dysrhythmia. These patients had lesions involving the anterolateral funiculus in the C2 segment, where respiratory fibers are intermingled with ascending pain fibers. Thus, these reports clearly document Ondine’s curse as a sequela of high cervical spinal cord lesions.
Spinal Trauma
OSA has been described in patients with cervical spine fractures [497] or high spinal cord injury [498, 499]. Guilleminault [500] described eight victims of neck trauma who showed sleep apnea, hypoxemia, and EDS and suggested that mild compression of the lower medulla and upper cervical spinal cord might cause respiratory disturbances during sleep after severe whiplash injury or odontoid fractures. In another report, Stockhammer et al. [501] documented sleep apnea syndrome in 55 % men and 20 % of women in a series of 50 randomly selected patients with tetraplegia resulting from spinal cord injuries. The authors concluded that the incidence of sleep apnea syndrome is high in tetraplegia, especially in older men with large neck circumference and long-standing spinal cord injuries. More recent reports also emphasized a high prevalence of sleep dysfunction and sleep-disordered breathing (SDB) [502–506] in spinal cord injury. Sleep complaints are frequent in spinal cord injury patients and may include difficulty in falling asleep, repeated awakening, impaired sleep quality, increasing use of sleeping medication, snoring, and daytime napping [503, 507]. Proserpio et al. [502] in a prospective observational study evaluated 35 patients with spinal cord injury (15 tetraplegics and 20 paraplegics) within the first year after injury. They noted OSA in nine (25.7 %) and PLMS in 10 (28.6 %) patients. In their patients, the frequency of OSA was higher in tetraplegics than paraplegics, similar to high prevalence of SDB in tetraplegics reported previously by Berlowitz et al. [508]. Another important observation in this study is the high prevalence of OSA after cervical cord injury. Finally, the absence of daytime sleepiness related to OSA in their patients is in line with similar previous observation [505] and that noted in heart failure patients with SDB [507].
There are a few scattered reports of PLMS associated with paraplegia due to spinal cord injury or other spinal cord lesions [509–512]. The authors of these papers concluded that the PLMS resulted from disinhibition of the spinal locomotor generator. It should be noted that the question of the spinal cord as the generator for PLMS remains highly speculative and controversial. In a recent preliminary neurophysiologic study in a subgroup of spinal cord injury patients, Ferri et al. [513] observed a cerebral and autonomic response to leg movements in 45 % of patients with acute spinal cord injury suggesting the potential presence of surviving spinal or extraspinal connections.
Sleep and Breathing Disturbances in Neuromuscular Disorders
Sleep disturbances in neuromuscular disorders are most commonly due to respiratory dysrhythmias secondary to involvement of the respiratory pump, which includes the diaphragm, the intercostal and other accessory muscles of respiration, and the upper airway muscles (genioglossus, palatal, pharyngeal, laryngeal, hyoid, and masseter muscles). Adverse effects on the motor neurons, the phrenic and intercostal nerves, or the neuromuscular junctions of the respiratory and oropharyngeal muscles, and primary muscle disorders affecting these muscles are responsible for respiratory dysrhythmia and sleep dysfunction [91, –519]. The most common complaint is EDS resulting from repeated arousals and sleep fragmentation due to transient nocturnal hypoxemia and hypoventilation in addition to sleep-related respiratory dysrhythmias. Some patients, particularly those with painful polyneuropathies, muscle pain, muscle cramps, and immobility due to muscle weakness, may complain of insomnia. The most common neuromuscular disorders causing SDB and sleep dysfunction include motor neuron disease (ALS); myasthenia gravis, including myasthenic syndrome; acute inflammatory demyelinating polyradiculoneuropathies (Landry/Guillain–Barré/Strohl syndrome); muscular dystrophies, including myotonic dystrophy; inflammatory myopathies; and congenital myopathies (Box 41.4 and Fig. 41.23). Many of these conditions are treatable; others show relentless progression, but even in these conditions, quality of life may be improved with prolongation of the natural course of the illness by timely and adequate treatment of SDB. It is therefore important for physicians taking care of patients with neuromuscular disorders to have a basic understanding of these disorders and a high index of suspicion for SDB in such patients so that patients can be either referred to specialists or treated adequately in a timely manner.


Fig. 41.23
Types of neuromuscular disorders
Box 41.4 Common Neuromuscular Disorders Causing Sleep-Disordered Breathing and Sleep Dysfunction
Motor neuron disease (amyotrophic lateral sclerosis [ALS]),
Polymyositis and post-polio syndrome,
Myasthenia gravis,
Myasthenic syndrome,
Acute inflammatory demyelinating polyradiculoneuropathy (Landry–Guillain–Barré syndrome),
Muscular dystrophies including myotonic dystrophies types 1 and 2 (DM1 and DM2),
Inflammatory myopathies (polymyositis and dermatomyositis),
Congenital myopathies.
Sleep-disordered breathing is commonly associated with insidiously developing chronic respiratory failure in neuromuscular disorders, especially in the advanced stages, but is often unrecognized and untreated [91, 513–519]. The most common SDB in neuromuscular disorders is sleep-related, especially REM-related, hypoventilation. Both central and upper airway obstructive apneas also occur. Bulbar muscle weakness in many patients may cause increased upper airway resistance, but adequate studies have not been undertaken to identify its true prevalence in neuromuscular disorders. Paradoxical breathing (movements of the thorax and abdomen in opposite directions) may be seen in patients with upper airway OSA and upper airway resistance syndrome. This is different from paradoxical inward movement of the abdomen with epigastric retraction instead of protrusion during inspiration as seen in patients with diaphragmatic paralysis. Nocturnal hypoventilation giving rise to hypoxemia and hypercapnia during sleep in the initial stage of neuromuscular disorders causes chronic respiratory failure; the abnormal blood gases may later present even during the daytime.
Mechanism of SDB and Respiratory Failure in Neuromuscular Diseases
Respiratory failure is defined as an inability of the lungs to effectively exchange gas and maintain normal acid-base balance as a result of failure of the respiratory system anywhere from the medullary respiratory controllers to the chest bellows and the lungs, including the upper airways. As a result of this failure, there is reduction in Pao 2 and increased Paco 2. A Pao 2 of less than 60 mm Hg and a Paco 2 of more than 45 mm Hg at sea levels are commonly considered criteria for respiratory failure. Most neuromuscular disorders cause ventilatory failure, which is defined as inadequate alveolar ventilation with reduced tidal volume causing low Pao 2 and high Paco 2.
Respiratory failure in neuromuscular disorders begins during sleep, followed by gradual or relentless progression unless interrupted by ventilatory support at night. A variety of physiologic changes occur in the central control of breathing and in the respiratory muscles during sleep (see Chap. 11) that are responsible for initiating respiratory failure in sleep in patients with neuromuscular disorders. Additionally, there may be a comorbid upper airway obstruction not related to neuromuscular disorders. However, in many of these patients, the upper airway muscles are also affected, causing upper airway OSA. The accessory muscles of respiration maintain breathing during NREM sleep in patients with weakness of the diaphragm, the main muscle of ventilation; however, during REM sleep, there is hypotonia or atonia of these accessory muscles, and ventilation then depends exclusively on the diaphragm. Therefore, in patients with diaphragmatic weakness, ventilation is severely affected during REM sleep, causing REM hypoventilation. This is the first stage of respiratory failure in neuromuscular disorders (Fig. 41.24). As the disease advances to the second stage, the accessory muscles of respiration are affected severely, thus causing ventilatory disturbance during NREM sleep [513, 520]. In the final stage (stage 3) of neuromuscular disorders, ventilation is affected even during the daytime, causing altered blood gases (e.g., hypoxemia and hypercapnia) during wakefulness. Stage 2 of respiratory failure may be related to either progression of the neuromuscular disorder or superimposed intercurrent infection (e.g., pneumonia), or both.


Fig. 41.24
Stages of NMD
Several authors aimed at identifying daytime predictors of SDB and nocturnal hypoventilation and its onset [521–523]. These authors concluded that progressive ventilatory restriction in neuromuscular diseases correlates with respiratory muscle weakness and can be predicted from daytime lung and respiratory muscle function. Inspiratory vital capacity (IVC) and maximum inspiratory muscle pressure (PImax) are the two important predictors of the onset of respiratory failure [521, 522]. IVC of less than 60 % and PImax of less than 4.5 kPa predicted onset of REM hypoventilation, IVC of less than 40 % and PImax of less than 4.0 kPa predicted both REM and NREM hypoventilation, and IVC of less than 25 % and PImax of less than 3.5 kPa predicted daytime respiratory failure. However, Lyall et al. [523] and Morgan et al. [524] suggested that the noninvasive maximal sniff pressure measurement is more sensitive than vital capacity and static maximal inspiratory muscle pressure in patients with ALS and in assessing the risk of ventilatory failure. Carratu et al. [525] in a later preliminary study in 31 ALS patients confirmed that maximal sniff nasal inspiratory pressure (SNIP) lower than 60 CMH20 may be an early predictor of SDB in ALS. It has also been suggested that a significant fall of vital capacity from the erect to the supine position (70 % or less of the predicted value) indicates the presence of diaphragmatic weakness [526–528]. A fluoroscopy will confirm the weak movement of the dome of the diaphragm during inspiration. A serial blood gas determination is important in detecting impending respiratory failure. It should be remembered that a normal daytime Pao 2 and Paco 2 does not exclude REM-related hypoventilation.
In patients with weak respiratory muscles, regardless of cause, the waking breathing difficulties may worsen during sleep. While patients are awake, both voluntary and metabolic respiratory controls are intact and central respiratory neurons increase the rate of firing or recruit additional respiratory neurons to maintain ventilation adequately to drive weak respiratory muscles [91]. During sleep, however, voluntary control is absent and respiration is dependent entirely on the metabolic control system. Respiratory neurons are thus vulnerable during sleep, aggravating the ventilatory problems and causing more severe hypoventilation and even apneas and hypopneas. Functional impairment of the sensitivity of the central respiratory neurons, causing decreased metabolic respiratory control, may also give rise to apnea-hypopnea during both REM and NREM sleep. Oropharyngeal (upper airway) muscle weakness coupled with REM-related hypotonia or atonia of the muscles may contribute to possible upper airway OSA.
In summary, breathing disorders causing sleep-related hypoventilation in neuromuscular disorders may be related to the following factors:
- 1.
Weakness of the respiratory and chest wall muscles causing impaired chest bellows,
- 2.
Increased work of breathing due to altered chest mechanics and reduced forced vital capacity caused by weakness of the chest wall muscles and diaphragm so that breathing is less efficient,
- 3.
Hyporesponsive chemoreceptors, which may be secondarily acquired or related to altered afferent inputs from skeletal muscle spindles, causing functional alteration of the medullary respiratory neurons,
- 4.
Weakness of upper airway muscles that increases upper airway resistance, adding respiratory muscle load or even upper airway OSA from complete closure of the upper airway,
- 5.
Decreased minute and alveolar ventilation during sleep,
- 6.
REM-related marked hypotonia or atonia of all the respiratory muscles except the diaphragm, causing increased diaphragmatic workload,
- 7.
Respiratory muscle fatigue due to increased demand on the respiratory muscles during sleep, particularly REM sleep,
- 8.
Kyphoscoliosis secondary to neuromuscular disorders, causing extrapulmonary restriction of the lungs with impairment of pulmonary functions, breathlessness, sleep apnea, and hypoventilation,
- 9.
Failure of central control of ventilation,
- 10.
Alteration of respiratory reflexes in upper airway and lung receptors and arousal responses.
All these factors may lead to ventilatory failure in neuromuscular disorders initially at night in the early stage. As a result of alveolar hypoventilation and ventilation-perfusion mismatching, hypoxemia and hypercapnia occur, giving rise to chronic respiratory failure even during the daytime at an advanced stage of the illness (Fig. 41.24).
Clinical Approach
The initial approach to clinical diagnosis of acute respiratory failure, as may occur in patients with acute inflammatory demyelinating polyradiculoneuropathies (e.g., Guillain–Barré syndrome) or myasthenic crisis, is quite obvious. Patients may have irregular, rapid, shallow, or periodic breathing, intermittent cessation of breathing, and cyanosis. However, recurrent hypoventilation in neuromuscular disorders may present insidiously and may initially remain asymptomatic [514, 515, 529–531]. A high index of clinical suspicion is needed. Clinical clues include the presence of EDS, nocturnal restlessness, frequent unexplained arousals, daytime fatigue, shortness of breath, orthopnea (breathlessness in supine position), morning headache, intellectual deterioration and failure to thrive and declining school performance in children. Signs of impending cor pulmonale include insomnia, morning lethargy, headache, and unexplained leg edema. Patients with neuromuscular disorders manifesting these clinical features should be investigated to uncover nocturnal hypoventilation in order to prevent adverse consequences of chronic respiratory failure, such as congestive cardiac failure and cardiac arrhythmia. Special attention during physical examination should be paid to uncover bulbar and respiratory muscle weakness, use of accessory muscles of respiration, and paradoxical breathing. Neuromuscular disorders causing SDB, respiratory failure, and sleep disturbance include ALS or motor neuron disease, primary muscle diseases, acute and chronic inflammatory demyelinating polyneuropathies, hereditary sensorimotor neuropathy, phrenic neuropathy, and neuromuscular junctional disorders (Fig. 41.23).
Amyotrophic Lateral Sclerosis
Amyotrophic lateral sclerosis is the most common degenerative disease of the motor neurons in adults, affecting the spinal cord, brain stem, motor cortex, and corticospinal tracts. It is characterized by a progressive degeneration of both upper and lower motor neurons manifesting as a varying combination of lower motor neuron (e.g., muscle weakness, wasting, fasciculation, dysarthria, and dysphagia) and upper motor neuron (e.g., spasticity, hyper-reflexia, and extensor plantar response) signs. The readers are referred to the revised El Escorial and Awaji criteria for diagnosing ALS [532]. ALS can cause profound sleep disturbances associated with EDS as a result of repeated arousals and sleep fragmentation due to nocturnal hypoventilation, recurrent episodes of sleep apnea, hypopnea, hypoxemia, and hypercapnia. Insomnia related to other factors, such as decreased mobility, muscle cramps, anxiety, and difficulty in swallowing, may be present in some patients. SDB in ALS may result from weakness of the upper airway, diaphragmatic, and intercostal muscles due to involvement of the bulbar, phrenic, and intercostal motor neurons. In addition, degeneration of central respiratory neurons may occur, causing central and upper airway OSAs. Generally, respiratory failure in ALS occurs late, but occasionally this may be a presenting feature requiring mechanical ventilation [529, 533]. Diaphragmatic weakness resulting from the degeneration of phrenic neurons is noted frequently in ALS and is mainly responsible for nocturnal hypoventilation, initially during REM sleep [514, 515]. Newsom Davis’ group [534] described eight patients with diaphragmatic paralysis resulting from a variety of motor disorders. One of their patients had Kugelberg–Welander syndrome. The following features may be helpful in the diagnosis of diaphragmatic paralysis:
- 1.
Breathlessness and EDS, suggesting alveolar hypoventilation,
- 2.
Paradoxical inward movement of the abdomen with epigastric retraction instead of protrusion during inspiration,
- 3.
An elevated diaphragm on chest radiography and paradoxical movement or decreased excursion of the diaphragm on fluoroscopy,
- 4.
Documentation of a very sensitive measurement showing a lack of change in the transdiaphragmatic pressure during a maximum inspiration,
- 5.
Diaphragmatic electromyographic (EMG) findings,
- 6.
Respiratory function tests with the evidence of a restrictive pattern,
- 7.
Blood gases showing hypoxemia and hypercapnia, suggesting alveolar hypoventilation,
- 8.
Documentation of sleep-related breathing abnormalities on PSG.
Sleep-related respiratory dysrhythmia is a common complication of ALS causing central and obstructive sleep apneas, sleep hypoventilation, and respiratory failure associated with daytime hypersomnolence [514, 515, 535–538]. Overnight polygraphic recording documenting both central and upper airway obstructive apneas (Fig. 41.25) associated with severe oxygen desaturation, and alveolar hypoventilation taken from a PSG tracing of one of our (SC) ALS patients is shown here.


Fig. 41.25
Overnight polysomnographic recording from a patient with amyotrophic lateral sclerosis presenting with upper and lower motor neuron signs, including bulbar palsy, showing recurrent periods of central apneas many of which are prolonged, followed by irregular ventilatory cycles (resembling ataxic breathing; see Fig. 41.7) accompanied by severe oxygen desaturation and sleep hypoxemia during REM sleep. Top four channels represent the electroencephalogram. EEG international electrode placement system, ABDM abdominal breathing effort; CHIN submental electromyogram (EMG); EKG electrocardiogram; LOC left electrooculogram; LT.GAST left gastroenemius EMG; LT.TIBIA left tibialis EMG; ORONAS oronasal airflow; PFLOW nasal pressure recording for airflow; ROC right electrooculogram; RT.GAST right gastroenemius EMG; RT.TIBIA right tibialis EMG; SAO 2 %, oxygen saturation [%] by finger oximetry; SNORE snoring recording; THORAX thoracic breathing effort
Ferguson et al. [535] studied 18 ALS patients with mild-to-severe bulbar muscle involvement and 10 age-matched control subjects. Most patients complained of difficulty initiating and maintaining sleep. All patients and controls had overnight PSG study, and 13 ALS patients had a second night of PSG study. ALS patients had more arousals and stage changes per hour, more stage 1 NREM sleep, and shorter total sleep time than controls. ALS patients had mild SDB with greater AHI than controls. It is notable that SDB was similar in ALS patients with or without respiratory muscle weakness. The SDB consisted of REM-related nonobstructive and central apneas. In an early study, Minz et al. [536] described PSG findings in 12 ALS patients, six men and six women. Four patients had both central and obstructive apneas. Sleep structure was normal in eight, but the others had frequent awakenings.
Howard et al. [537] described 14 patients with motor neuron disease associated with respiratory dysfunction. Eleven received respiratory support, including CPAP and intermittent positive pressure ventilation (IPPV) at night with considerable benefit. In a recent report, Lo Coco et al. [538] studied 100 consecutive ALS patients and 100 age- and sex-matched control subjects using PSQ and ESS as well as overnight PSG in 12 patients and the ALS Functional Rating Scale—Review (ALSFRS-R) in all patients. Fifty-nine percent of patients and 36 % of controls had sleep disturbances. The most common nighttime sleep complaints by patients with ALS included nocturia (54 %), sleep fragmentation (48 %), and nocturnal leg cramps (45 %). PSG showed decreased sleep efficiency and fragmented sleep architecture. These authors demonstrated that ALS patients had significant impairment of quality of sleep which correlated with the severity of illness (reduced ALSFRS-R score), daytime somnolence, ESS score, and depression. This is an important study challenging the clinicians to be aware of sleep-wake problems in ALS patients, because timely intervention could lead to better quality of life for these patients.
There have been several reports focusing on the role of noninvasive IPPV at night on the quality of life and the prognosis (see later in the Sect. “Treatment of Sleep and Respiratory Dysfunction Secondary to Neurologic Disorders”).
Other Types of Motor Neuron Diseases
SDB causing sleep disturbance and daytime symptoms has also been noted in other types of motor neuron diseases, such as Kugelberg–Welander syndrome, a variant of juvenile-type motor neuron disease, as well as spinal muscular atrophy types 1 and 2 in children and adolescents [539–542].
Spinal muscular atrophy (SMA) consists of a group of recessively inherited neuromuscular disorders resulting from mutations of the survival motor neurons (SMN-1) gene on chromosome 5q [515]. Based on the age of presentation and the number of functioning copies of the SMN-2 gene, SMA has been divided into four subtypes: SMA-1 (acute infantile type also called Werdnig–Hoffman disease), SMA-2 (chronic infantile form), SMA-3 (juvenile form or Kugelberg–Welander disease), and SMA-4 (adult onset). Those with a greater number of copies of the SMN-2 gene begin later in life and have less severe symptoms. Nocturnal noninvasive IPPV eliminates SDB, improves sleep quality, and prolongs survival in children with SMA types 1 and 2 [539, 541, 542].
Polyneuropathies
The cardinal manifestations of polyneuropathies are bilaterally symmetric, distal sensory symptoms and signs, and muscle weakness and wasting (affecting the legs more often than the arms). Peripheral neuropathies may be caused by a variety of heredofamilial and acquired diseases. Disorders of the phrenic, intercostal, and other nerves supplying the accessory muscles of respiration can cause weakness of the diaphragm, intercostal, and accessory respiratory muscles, giving rise to breathlessness on exertion, hypoxia, and hypercapnia. These respiratory dysrhythmias become worse during sleep. Sleep disturbances in polyneuropathies may result from painful neuropathies, partial immobility owing to a paralysis of the muscles, or SBD.
Acute inflammatory Neuropathy
The most common cause of respiratory dysfunction in polyneuropathy is acute inflammatory demyelinating polyradiculoneuropathy (Landry–Guillain–Barré–Strohl syndrome). The characteristic clinical manifestations consist of predominantly motor deficits associated with rapidly progressive ascending paralysis beginning in the legs and manifesting maximally in 2–3 weeks. In approximately 20–25 % of cases, severe respiratory involvement has been reported, and the critical period is usually the first 3–4 weeks of the illness. It is important to recognize and treat the ventilatory dysfunction. Even the mild respiratory dysrhythmia during wakefulness may worsen during sleep, causing sleep apnea and hypoventilation. Patients with Guillain–Barré syndrome (GBS) may also have vivid dreams and hallucinations with abnormalities of sleep structure in the form of sleep-onset REM, REM sleep without atonia, RBD, and autonomic dysfunction, which have been described in approximately one-third of the GBS patients admitted to an intensive care unit [543]. In a prospective questionnaire-based study [544], more than half of GBS patients had sleep disturbance characterized by delayed sleep onset, sleep fragmentation, and decreased total sleep time, particularly during the first week of hospitalization with subsequent improvement. The question of long-term persistence of SDB in GBS remains undecided until further research [545].
Charcot–Marie–Tooth Disease
Charcot–Marie–Tooth (CMT) disease is the most common inherited neuropathy which has been separated into two main groups: a demyelinating form (CMT1) and an axonal form (CMT2) which has several subtypes (e.g., CMT2A…CMT 2G). Patients with CMT 2C are especially prone to develop vocal cord palsy, possibly due to laryngeal nerve involvement, as well as diaphragmatic dysfunction, hypoventilation, and sleep apnea [546], presumably due to phrenic neuropathy. Sleep dysfunction in CMT results mainly from comorbid sleep apnea [547–552] which may occur in both CMT1 and CMT2 subtypes and restless legs syndrome/Willis–Ekbom disease (RLS/WED) [549, 553–558].
RLS/WED, Polyneuropathies, and Sleep Dysfunction
RLS is seen most commonly in CMT2 but has also been noted in CMT1 type. Gemignani et al. [553, 555–558] observed that RLS associated with sleep dysfunction was more prevalent in subgroups of patients with painful polyneuropathies (both retrospective and prospective studies) compared with controls, suggesting small fiber neuropathy and central sensitization. In contrast, Lim et al. [559] based on their studies of 56 patients with primary RLS/WED and 36 age- and sex-matched controls, using quantitative sudomotor axon reflex test (QSART) and sensory testing concluded that abnormal sensory perception in RLS/WED patients resulted from an impairment of central somatosensory processing rather than small fiber neuropathy.
Other Neuropathies and Sleep Dysfunction
In addition to GBS, a high prevalence of RLS/WED with sleep dysfunction has been described also in chronic inflammatory demyelinating polyneuropathy (CIDP) [557, 560, 561] and those with painful polyneuropathies [556, 558, 559]. On rare occasion, multifocal motor neuropathy (MMN), a distinctive acquired demyelinating neuropathy with conduction block, may present with sleep hypoventilation (presumably due to bilateral phrenic neuropathy) which may be successfully treated with a combination of intravenous immunoglobulin (IVIG) and nocturnal bilevel positive ventilation therapy [562]. OSA associated with chronic sleep dysfunction itself may be a risk factor for axonal polyneuropathy due to recurrent intermittent hypoxemia [563, 564].
Phrenic neuropathies of diverse etiologies associated with neuropathies and plexopathies may cause SDB and sleep difficulty as a result of diaphragmatic dysfunction [514, 515]. Trauma, inflammatory polyneuropathy, and infiltrative lesions (e.g., neoplasms) may cause phrenic neuropathy. Phrenic nerve injury causing SDB with sleep dysfunction has also been noted as a complication of cardiothoracic surgeries [515, 565].
Primary Muscle Diseases (Myopathies)
Myopathies are primary muscle disorders characterized by weakness and wasting of the muscles resulting from a defect in the muscle membrane or the contractile elements that is not secondary to a structural or functional derangement of the lower or upper motor neurons [91, 514]. The characteristic clinical presentation consists of symmetric, proximal muscle weakness and wasting in the upper or lower limbs without sensory impairment or fasciculations. The causes include hereditary muscular dystrophies with or without myotonia; glycogen storage diseases; myoglobinuric myopathies; congenital nonprogressive myopathies with distinct morphologic characteristics; and various acquired metabolic, inflammatory, and noninflammatory myopathies. Some of these patients may report breathing disorders during sleep or worsening of the respiratory dysfunction during sleep. Generally, respiratory disorders show manifestations in the advanced stage, but a small number of patients may present with respiratory failure at an early stage. In many such patients, the true incidence of the sleep disturbances and sleep-related respiratory dysrhythmias cannot be determined without a systematic PSG study. Factors responsible for breathing disorders associated with hypoventilation and sleep apnea in these patients may be summarized as follows [91, 514, 515]: impairment of chest bellows owing to weakness of the respiratory and chest wall muscles, increased work of breathing, and functional changes in the medullary respiratory neurons that could be due to hyporesponsive or unresponsive chemoreceptors acquired secondarily. The other suggestion for carbon dioxide hyposensitivity is altered afferent input from the skeletal muscle receptors [528]. Sleep disturbances generally occur in muscle disorders secondary to sleep-related respiratory dysrhythmias. Alveolar hypoventilation, both during wakefulness and sleep, should be diagnosed early in these patients to prevent dangerous or fatal hypoventilation during sleep or during administration of drugs, general anesthetic agents, and respiratory infections [91, 514]. Complaints of daytime hypersomnolence and breathlessness should direct attention to the possibility of SDB in these patients.
Muscular Dystrophy
The most common type of muscular dystrophy (nonmyotonic) associated with respiratory dysrhythmia-related sleep dysfunction is Duchenne’s muscular dystrophy (DMD) and its variant, Becker muscular dystrophy (BMD). DMD is an x-linked recessive disorder with mutation of the dystrophin gene causing sarcolemmal membrane instability. Dystrophin is absent in DMD but is reduced in BMD. DMD is a relentlessly progressive disorder of muscle weakness, involving initially the pelvifemoral muscles resulting in an inability to stand and walk with confinement to a wheel chair within a few years. Respiratory failure with repeated awakenings at night and EDS occur in the late stage of the disease associated with alveolar hypoventilation and obstructive sleep apnea (OSA). Hypoventilation results from respiratory muscle weakness and scoliosis with restrictive pulmonary deficit, whereas OSA is secondary to upper airway muscle weakness.
Sleep-related respiratory dysrhythmias (both obstructive and central apneas) accompanied by O2 desaturation and daytime hypersomnolence have been described in many patients with Duchenne’s muscular dystrophy [517, 566–571]. Although respiratory failure is most commonly noted in Duchenne’s muscular dystrophy, sometimes it also occurs in the more advanced stages of Becker’s, limb-girdle, and facioscapulohumeral muscular dystrophies [514, 515, 517].
Smith and associates [567] described 14 patients with Duchenne’s muscular dystrophy who had sleep apneas or hypopneas associated with marked O2 desaturation. These authors stated that the severity of SDB in Duchenne’s muscular dystrophy could not reliably be ascertained from daytime pulmonary function studies and asserted that sleep studies are essential.
Howard et al. [568] described nocturnal hypoventilation and respiratory failure in 84 patients with primary muscle disorders that included Duchenne’s, Becker’s, limb-girdle, and facioscapulohumeral muscular dystrophies; adult-onset acid maltase deficiency; myotonic dystrophy; polymyositis; congenital myopathies; and rigid spine syndrome. All patients needed ventilatory support in the form of negative or positive pressure ventilation or tracheostomy.
Khan and Heckmatt [569] studied 21 patients aged 13–23 years with Duchenne’s muscular dystrophy and 12 age-matched controls using 2 consecutive nights of PSG. They noted apneas, 60 % of which were obstructive in nature, with hypoxemia in 12 patients. The respiratory care of patients with Duchenne’s muscular dystrophy has been summarized in a consensus statement developed by the American Thoracic Society [534].
In a more recent study, Polat et al. [572] studied 35 children (12 with DMD and 23 controls) using PSG and they noted sleep-wake-related symptoms in 50 % of their patients. In an earlier study [517], similar symptoms were seen in up to 42 % and OSA in 16.6 %. These authors concluded that being wheelchair-bound or having scoliosis did not predict SDB, and so these patients should be investigated with PSG recording because if DMD patients are found to have SDB, treatment with nocturnal noninvasive positive pressure ventilation will improve the quality of life and longevity. In one of the largest studies [573] of 32 children with DMD by Suresh et al., OSA was noted in 31 % and these authors also recommended PSG for wheelchair-bound patients. They noted a bimodal presentation with OSA occurring in the first decade, whereas hypoventilation was seen more commonly in the second decade.
SDB including hypoventilation and OSA has been described in limb-girdle muscular dystrophy [574–576] (several subtypes are described based on distinct molecular abnormalities) and facioscapulohumeral muscular dystrophy [577, 578], the third most common form of muscular dystrophy after DMD and myotonic dystrophy, but not in a systematic manner.
Khan et al. [579] described eight children 6–13 years of age with congenital myopathy, congenital muscular dystrophy, and rigid spine syndromes with respiratory failure. PSG documented nocturnal hypoxemia and severe hypoventilation. Sleep disturbances included repeated awakenings. Sleep complaints and sleep-related periodic respiratory dysrhythmias are also common in other congenital myopathies such as nemaline rod myopathy, centrotubular and central core disease, merosin deficiency myopathy, and congenital muscular dystrophy [579–584]. Most of these present in childhood, but sometimes there is delayed presentation in adulthood [579–581] (see further on).
Myotonic Dystrophy
Dystrophica myotonica, or myotonic dystrophy type 1 (DM1), is an adult-onset, dominantly inherited muscular dystrophy associated with myotonia. DM1 is a multisystem disorder due to a trinucleotide expansion mutation (CTG sequence) on the DMPK gene on chromosome 19, frontal baldness, cataract, endocrinopathy, and neuropsychologic deficit. Benaim and Worster-Drought [585] were most probably the first to describe alveolar hypoventilation in myotonic dystrophy. Alveolar hypoventilation associated with hypoxemia, as well as impaired hypercapnic and hypoxic ventilatory responses, may be present in both the early and the late stages of the illness. Several authors [517, 586–590] performed polygraphic studies that showed central, mixed, and upper airway obstructive sleep apneas, and sleep-onset REM was noted in some patients. The latter finding may have been due to sleep deprivation secondary to sleep-related respiratory disturbances. Two fundamental mechanisms account for the SDB in this illness: (1) weakness and myotonia of the respiratory and upper airway muscles and (2) an inherited abnormality of the central control of ventilation, most likely related to a common generalized membrane abnormality of the muscles and other tissues, including brain stem neurons that regulate breathing and sleep [587–591].
Sleep studies by Bye et al. [464] in four patients with myotonic dystrophy showed REM sleep-related O2 desaturation and sleep disorganization. Several other authors have described alveolar hypoventilation, daytime somnolence, and periodic breathing in patients with myotonic dystrophy in single case reports and small and large series [517, 587–604].
Begin et al. [599] found a high prevalence of chronic alveolar hypoventilation in a series of 134 patients with myotonic dystrophy. The authors suggested that the central ventilatory control mechanism is abnormal in myotonic dystrophy patients, contributing to chronic alveolar hypoventilation. These authors concluded that the chronic alveolar hypoventilation resulted from a combination of inspiratory muscle weakness and loading. In addition, the presence of EDS suggested reduced central ventilatory drive or sleep apnea in these patients. The clinicopathologic study by Ono et al. [602] of one patient with myotonic dystrophy, alveolar hypoventilation, and hypersomnia supported the hypothesis postulated by Begin et al. [599]. On postmortem examination of this patient, Ono et al. [602] observed significant neuronal loss and gliosis in the midbrain and pontine raphe, as well as the pontomedullary reticular formation.
Park and Radtke [601] reviewed seven patients with myotonic dystrophy referred to their sleep disorder center. All patients had PSG. Five patients were subsequently given an MSLT. Each of the five who had an MSLT showed the evidence of moderate hypersomnia. Three of these five patients had two sleep-onset REM episodes, only one of whom showed the evidence of sleep apnea in the overnight PSG study. Human leukocyte antigen (HLA) typing was negative for DQW2, but DQW1 was present in two patients. The authors reviewed the literature available (they published their own paper in 1995) and found 86 patients, including their seven patients, with myotonic dystrophy who also had hypersomnolence. Ten percent of the reported patients with hypersomnolence had documented alveolar hypoventilation. Respiratory center hypoexcitability or myotonic muscle weakness is thought to be responsible for alveolar hypoventilation. Correction of hypoventilation does not always lead to improvement of EDS [597, 601]. SDB events were noted in 57 % of the reported patients with EDS, and both central and obstructive sleep apneas were observed. The presence of sleep-onset REMs in these patients supported the hypothesis of a primary CNS abnormality as the cause of EDS [601]. EDS in myotonic dystrophy patients often occurs in the absence of sleep apnea [600, 601]. EDS in their patients [601] responded to methylphenidate treatment. Martinez-Rodriguez et al. [603] measured CSF hypocretin-1 levels in six patients with myotonic dystrophy type 1 complaining of excessive daytime sleepiness who were HLA-DQB1*0602 negative and found to have significantly lower hypocretin-1 levels compared to the control values. The authors concluded that the dysfunction of the hypothalamic hypocretin system may be responsible for hypersomnia in myotonic dystrophy type 1. However, other authors [604, 605] did not find low CSF hypocretin levels in DM1 patients.
In summary, SDB including OSA and nocturnal hypoventilation, EDS, and REM sleep dysregulation are frequently noted in DM1 patients. There is reasonable explanation for the mechanism of sleep-related breathing disorder as stated above. However, an explanation for REM sleep dysregulation and EDS is not always obvious. In a case-control study including 40 DM1 patients and 40 controls, Yu et al. [590] observed EDS in 80 % of patients (ESS score was more than 10 in 31.4 %, and MSLT mean latency was less than 8 min in 12.5 %) and one SOREM in the majority of the patients, whereas two or more SOREMs were seen in about one-third of the patients. These authors also found higher REM density in patients than in controls. REM sleep dysregulation with two or more SOREMs or increased REM density in DM1 patients was also noted by Park et al. [601], Martinez-Rodriguez and coinvestigators [603], and Gibbs et al. [606]. It has been suggested that EDS and REM sleep dysregulation in DM1 patients are not necessarily related to SDB but may be due to an intrinsic CNS dysfunction involving sleep-wake-regulating neurons [590, 593, 600, 601, 604, 607].
Sleep disturbances have also been reported in proximal myotonic myopathy (PROMM), also known as myotonic dystrophy type 2 (DM2). PROMM is an autosomal dominant multisystem disorder caused by a CCTG repeat expansion in intron 1 of the zinc finger protein 9 gene (ZNF9), which is differentiated from myotonic dystrophy type 1 by the absence of a chromosome 19 CTG trinucleotide repeat that is associated with type 1 [608, 609].
DM2 has many overlapping clinical features with DM1 such as cataracts, myotonia, cardiomyopathy, autosomal dominant inheritance, diffuse muscle aches and pains, and insulin resistance, but differs from DMI in more proximal distribution of muscle weakness, later onset of the illness, slower progression, and genetic testing. Sleep disturbances including SDB and hypersomnolence have been described in many DMI patients (see above), but a description of sleep problems has been characteristically absent in DM2 until recently. In a brief report, the senior author described sleep disturbances in two sisters, aged 51 and 53, with PROMM [610, 611]. The patients had difficulty initiating sleep, EDS, snoring, frequent awakenings, and movements during sleep. Overnight PSG study showed decreased sleep efficiency, increased number of arousals, and sleep architecture abnormalities. One patient had absent REM sleep, and the other patient had dissociated REM sleep characterized by phasic REM bursts associated with EEG patterns showing sleep spindles and alpha intrusions. These sleep abnormalities in PROMM suggested involvement of the REM-NREM-generating neurons as part of a multisystem membrane disorder. Subsequently, our (SC) group reported more patients with DM2 showing SDB [612] and one with REM behavior disorder [613]. Many other investigators in case series and scattered case reports of DM2 reported a variety of sleep dysfunction including SDB, PLMS, EDS, RLS, and insomnia [614–618]. However, no systematic study involving a large number of DM2 patients with longitudinal follow-up is available. The MRI findings of white matter hyperintensity in T2-weighted images in six patients from three families with PROMM described by Hund et al. [619] suggested brain involvement in PROMM, but the relationship between the sleep disturbances and the MRI abnormalities remains to be determined.
Acid Maltase Deficiency and Other Glycogen Storage Disorders
Alveolar hypoventilation has been described in several cases of mild-to-moderate myopathy associated with adult-onset acid maltase deficiency, a variant of glycogen storage disease, also known as Pompe disease. Pompe disease (or glycogen storage disease type II) is an autosomal recessive, serious, and often fatal disorder of glycogen metabolism. It is due to mutations in the acid alpha-glucosidase (GAA) gene, absence of which causes cardiorespiratory failure in the first year, whereas reduced GAA activity gives rise to progressive respiratory failure later in life [620, 621]. The disease may present in the early stage with diaphragmatic weakness causing hypoventilation, initially during REM sleep and later causing respiratory failure even during the daytime. Presence of macroglossia and significant tongue weakness should direct attention to upper airway dysfunction causing OSA. It is important to make the correct diagnosis early by performing electromyographic (EMG), biochemical, histochemical, or morphological examination of muscle biopsy samples, determination of low GAA activity in skin fibroblasts or blood samples, respiratory function, and genetic testing as specific treatment is now available. The Federal Drug Administration (FDA) in the USA and the European Authority recently approved alglucosidase alfa (recombinant human GAA, Myozyme, Genzyme Corporation, Cambridge, MA, USA) as enzyme replacement therapy (ERT) for the treatment of Pompe disease (biweekly intravenous infusion of 20 mg/kg body weight) [620, 621]. Subsequent clinical data suggest that ERT delays progression of symptoms, but many patients will require additional ventilator support, especially during sleep [620].
Bye et al. [464] described REM sleep-related hypoxemia and sleep disorganization in acid maltase deficiency. An adult patient with acid maltase deficiency with severe OSA and respiratory failure was reported by Margolis et al. [622]. Despite ventilatory support, the patient died. At postmortem examination, profound muscle replacement by fibrofatty tissue was noted in the tongue and diaphragm. The authors suggested that severe tongue weakness due to fatty metamorphosis associated with macroglossia contributed to the upper airway obstruction in this patient. The brain was not examined at the autopsy.
Other Varieties of Congenital Myopathies
Riley et al. [566] described alveolar hypoventilation in two patients with congenital myopathies (one with nemaline myopathy and the other with a myopathy of uncertain type). The patients’ ventilatory response to carbon dioxide was absent, and the authors suggested that the alveolar hypoventilation may have been due to a primary defect in the central chemoreceptor control of breathing. Their other suggestion was that the sensory stimuli from skeletal muscle receptors (e.g., muscle spindles) may have played a role in the blunted hypercapnic ventilatory response by altering afferent input to the CNS. There are other reports of sleep hypoventilation in congenital myopathy [464, 579, 622– 624].
Miscellaneous Myopathies
Obstructive sleep apnea with sleep-related hypoxemia and sleep disturbances have also been described in patients with polymyositis [464, 517, 568, 625] including inclusion body myositis [626] and mitochondrial encephalomyopathy [627–630].
Neuromuscular Junction Disorders
Myasthenia gravis, myasthenic syndrome, botulism, and tick paralysis are several neuromuscular junction disorders characterized by easy fatigability of the muscles, including the bulbar and other respiratory muscles, owing to failure of neuromuscular junctional transmission of the nerve impulses. The most important of these conditions is myasthenia gravis, an autoimmune disease characterized by a reduction in the number of functional acetylcholine receptors in the postjunctional region. Acute respiratory failure is often a dreaded complication of myasthenia gravis, and patients need immediate assisted ventilation for life support [91, 514, 515]. The respiratory failure, moreover, may be mild during wakefulness but may deteriorate considerably during sleep.
An important study by Quera-Salva et al. [631] reported the pulmonary function and PSG studies of 16 women and 4 men whose mean age was 40 years and who were diagnosed and treated for myasthenia gravis. Polysomnographic findings included moderately disturbed nocturnal sleep with an increase in stage 1 NREM and decreased slow-wave and REM sleep. Eleven patients had an RDI of 5 or higher. They had central, obstructive, and mixed apneas and hypopneas accompanied by decreased O2 saturation. All patients with REM sleep-related apneas or hypopneas had disturbed nocturnal sleep with a sensation of breathlessness. Twelve patients had insufficient sleep owing to awakening in the middle of the night and early morning hours with a sensation of breathlessness. Four of the 12 patients also had daytime hypersomnolence. The authors suggested that those patients of advancing age, moderately increased BMI, abnormal pulmonary function results, and daytime abnormal blood gas concentrations are at particular risk for SDB. Since this report, there have been a few other reports of SDB in patients with myasthenia gravis [632–635].
Manni et al. [632] studied breathing patterns during sleep in 14 patients with mild generalized myasthenia gravis. Polysomnographic study documented infrequent central apneas, mainly during REM sleep, in five patients associated with O2 desaturation. Putman and Wise [633] described a 54-year-old woman with myasthenia gravis and episodes of shortness of breath, which were more severe at night. The flow-volume loops suggested extrathoracic airway obstruction. They also surveyed a total of 61 myasthenia gravis patients referred to their pulmonary function laboratory during 42 months. They found a pattern of extrathoracic upper airway obstruction in 7 of the 12 patients who had flow-volume loops. Nicolle et al. [634] randomly selected 100 patients with myasthenia gravis out of 400 patients from their database. They found a prevalence of OSA of 36 % based on PSG study in 50 patients compared to an expected prevalence of 15–20 % of the general population. The prevalence of OSAS (those with daytime sleepiness) was 11 % compared to 3 % in the general population. The authors suggested that it is important to inquire about OSA symptoms in myasthenia gravis patients for adequate management of fatigue in these patients. Prudlo et al. [635] investigated sleep and breathing in 19 myasthenia gravis patients utilizing two consecutive overnight PSG studies. These authors found clinically relevant SDB in terms of OSA (defined as an RDI of > 0/h) in only four patients, who had also a few central apneas. The authors failed to confirm the high occurrence of central respiratory events during sleep and also failed to find a causal relationship between medically stable myasthenia gravis and OSA. Thus, some investigators observed more central than obstructive apneas [632], whereas others noted predominantly obstructive apneas. The final answer remains to be determined. Some investigators [636] reported a high prevalence of patient-reported sleep disturbance which was correlated with disease severity, while some other researchers [637] reported a high prevalence of RLS in MG compared with controls.
Fernandes Oliveira and coinvestigators [638] made a meta-analysis of 17 cross-sectional observational or clinical studies assessing the quality of sleep in MG patients published between 1970 and 2014. They found that the literature is limited and the evidence for sleep dysfunction in MG is inconclusive because some studies reported poor sleep quality, EDS, RLS, and SDB in patients with MG, whereas others did not report such associations. The authors recommended further research to investigate sleep dysfunction in MG.
Myasthenic syndrome, or Lambert–Eaton syndrome, is a disorder of the neuromuscular junction in the presynaptic region and is often a paraneoplastic manifestation, mostly of oat cell carcinoma of the lungs. Patients complain of muscle weakness and fatigue involving the limbs accompanied by decreased or absent muscle stretch reflexes and characteristic electrodiagnostic findings that differentiate this from myasthenia gravis. Very few data exist on SDB and sleep dysfunction in Lambert–Eaton myasthenic syndrome.
Botulism caused by Clostridium botulinum and tick paralysis caused by the female wood tick Dermacentor andersonii may also cause neuromuscular junctional transmission defects, which are due to released toxin, and may cause respiratory failure and sleep dysfunction, but adequate studies have not been performed.
Sleep and Breathing Disorders in Autonomic Failure
Anatomically and functionally, sleep, breathing, and the autonomic nervous system (ANS) are closely interrelated [91, 639–644]. To understand the sleep and breathing disorders in autonomic failure, it is important to understand the functional anatomy of sleep, control of breathing, and the central autonomic network. A brief review of the functional anatomy of sleep is given in the beginning of this chapter, and the neurophysiology of sleep is also described extensively in Chaps. 5 and 8. Functional neuroanatomy of respiration, control of breathing during sleep and wakefulness, and the central autonomic network with its integration of sleep and breathing are reviewed briefly in Chap. 11.
In all of these conditions, respiratory muscles may be affected and patients may require assisted ventilation. They can exhibit sleep hypoventilation and sleep apnea. Profound functional changes occur during sleep in circulation, respiration, thermoregulation, and the gastrointestinal and urogenital systems due to alterations in autonomic outflow [639, 640] (see also Chap. 11). Thus, sleep has an important effect on the functions of the ANS, and dysfunction of the ANS may have significant impact on human sleep. Sleep and breathing disorders have in fact been described in many patients with autonomic failure. It is also important to remember that the peripheral respiratory receptors, the central respiratory neurons, and the hypnogenic neurons in the preoptic-hypothalamic area and the region of the NTS in the medulla are intimately linked by the ANS, making it easy to comprehend why sleep and breathing disorders should be associated with autonomic failure.
Autonomic failure (AF) may be classified into primary and secondary types. Primary autonomic failure (without known cause) includes pure autonomic failure without any somatic neurologic deficits (Bradbury–Eggleston syndrome), multiple system atrophy (MSA) formerly known as Shy–Drager syndrome, postural tachycardia syndrome (PoTS), familiar dysautonomia, and autoimmune autonomic neuropathy or acute pan-dysautonomia. The most well-known condition with autonomic failure in which sleep and respiratory disturbances have been reported and well described is MSA with progressive autonomic failure. In a consensus statement [645] in 1996 sponsored by the American Autonomic Society and the American Academy of Neurology, multiple system atrophy is the favored term, replacing Shy–Drager syndrome. MSA defines a sporadic, adult-onset progressive disorder characterized by autonomic dysfunction, parkinsonism, and ataxia in any combination. Striatonigral degeneration is the term used when the predominant feature is parkinsonism. The term sporadic olivopontocerebellar atrophy is used when cerebellar features are present. Finally, when autonomic failure is the predominant feature, the term Shy–Drager syndrome is often used. Familial dysautonomia, a recessively inherited primarily autonomic failure, is also known to be associated with disturbances of breathing and sleep. Congenital central hypoventilation syndrome (CCHS) (see Chap. 33), another autosomal dominant familial disorder due to mutations of PHOX 2B gene, has recently been considered to be a disorder of autonomic regulation, although classified in the ICSD-3 [101] under the category of “Sleep-related Hypoventilation Disorders.” A large number of neurologic and general medical disorders are associated with prominent secondary autonomic failure. In many patients with diabetic autonomic neuropathies, amyloid neuropathy, and GBS, sleep and sleep-related respiratory disturbances have been noted. In many neurologic conditions, sleep and respiratory disturbances are secondary to the structural lesions involving the central hypnogenic or respiratory neurons. Some examples of neurodegenerative diseases with autonomic failure and sleep dysfunction are PD (see Chap. 39) and DLBD with dementia (see earlier section) and fatal familial insomnia (FFI), a rare prion disease with severe sleep disturbances and dysautonomia (see later in this chapter). Finally, some primary sleep disorders, such as chronic insomnia disorder, narcolepsy-cataplexy and upper airway OSAS, a very common primary sleep disorder, may be associated with autonomic deficits.
Multiple System Atrophy with Progressive Autonomic Failure (Shy–Drager Syndrome)
In 1960, Shy and Drager [646] described a neurodegenerative disorder characterized by autonomic failure and MSA. Since their description, there have been many reports [87, 91, 99, 640, 647–660] of the condition, which has generally come to be known as Shy–Drager syndrome or multiple system atrophy with progressive autonomic failure. MSA defines a sporadic adult-onset progressive disorder of multiple systems characterized by autonomic dysfunction, parkinsonism, and cerebellar ataxia in various combinations (Box 41.5). A second consensus conference held in 2007 published new diagnostic criteria for definite, probable, and possible MSA (Box 41.6) [656].
Box 41.5 Salient Clinical Manifestations of Multiple System Atrophy [77]
A. Autonomic Features
- 1.
Cardiovascular
Orthostatic hypotension,
Postprandial hypotension,
Postural syncope,
Postural dizziness, faintness, or blurring of vision,
Orthostatic intolerance.
- 2.
Genitourinary
Urinary bladder dysfunction (incontinence, hesitancy, frequency, nocturia),
Impotence in men.
- 3.
Sudomotor
Hypohidrosis or anhidrosis,
- 4.
Gastrointestinal
Gastroparesis,
Intermittent diarrhea or constipation (intestinal or colonic dysmotility),
Abnormal swallowing (esophageal dysmotility).
- 5.
Ocular
Horner’s syndrome,
Unequal pupils.
B. Nonautonomic Manifestations
- 1.
Parkinsonism
Rigidity,
Bradykinesis or akinesis,
Postural instability.
- 2.
Cerebellar dysfunction
Ataxic gait,
Scanning speech,
Dysmetria,
Dysdiadochokinesia,
Intention tremor.
- 3.
Upper motor neuron signs
Extensor plantar responses,
Hyper-reflexia
Spasticity.
- 4.
Lower motor neuron signs
Muscle wasting,
Fasciculations.
- 5.
Respiratory
Sleep apnea-hypopnea,
Other respiratory dysrhythmias,
- 6.
REM sleep behavior disorder
- 7.
Cognitive decline (5–26 %)
- 8.
Normal sensation.
Box 41.6 Criteria for the Diagnosis of Multiple System Atrophy (MSA) Based on Second Consensus Statement [617]
Definite MSA
A sporadic, progressive adult-onset (>30 years of age) disease with neuropathologic demonstration of α-synuclein-positive glial cytoplasmic inclusions in the CNS,
Evidence of neurodiagnostic changes in striatonigral or olivopontocerebellar structures.
Probable MSA
A sporadic, progressive adult-onset (>30 years of age) disease with the evidence of autonomic dysfunction (e.g., orthostatic fall of blood pressure by at least 30 mm Hg systolic or 15 mm Hg diastolic within 3 min of standing, urinary incontinence, or erectile dysfunction in men)
Parkinsonian features (e.g., bradykinesia, rigidity, tremor, and postural instability) that are poorly levodopa responsive, or
Cerebellar features (e.g., gait and limb ataxia, scanning dysarthria, and cerebellar type of oculomotor dysfunction).
Possible MSA
A sporadic, progressive adult-onset (>30 years of age) disease with parkinsonian features or cerebellar features or
evidence of at least one autonomic dysfunction (orthostatic fall of blood pressure should be significant, which may not meet the level required for probable MSA).
Presence of at least one of the following additional features (either a clinical or a neuroimaging abnormality):
stridor and hyper-reflexia with Babinski sign.
Possible MSA-P
Parkinsonian or cerebellar features; dysphagia within 5 years of motor onset; olivopontocerebellar or putaminal atrophy on brain MRI; FDG-PET hypometabolism in putamen, brain stem, or cerebellum.
Possible MSA-C
Cerebellar features; presynaptic nigrostriatal dopaminergic denervation on SPECT or PET scan; olivopontocerebellar or putaminal atrophy on MRI; FDG-PET hypometabolism in putamen, brain stem, or cerebellum.
CNS central nervous system; FDG-PET fluorodeoxyglucose positron-emission tomography; MRI magnetic resonance imaging; PET positron-emission tomography; SPECT single-photon-emission computed tomography.
Patients frequently manifest sleep and respiratory disturbances. Initially, they present with autonomic failure of both the sympathetic and the parasympathetic systems. They may present with symptoms related to orthostatic hypotension (e.g., postural dizziness and faintness or even frank loss of consciousness in the erect posture), urinary sphincter dysfunction (e.g., frequency, urgency, hesitancy, dribbling, and overflow incontinence), hypohidrosis or anhidrosis, and impotence in men. After 2–6 years, patients lapse into the second stage, showing some combination of cerebellar, extrapyramidal, upper motor neuron, and lower motor neuron dysfunction, including bulbar deficits. Most patients manifest a parkinsonian-cerebellar syndrome. In some, atypical parkinsonian features (e.g., bradykinesia, rigidity, and postural instability) predominate; in others, pancerebellar dysfunction predominates. There has been an increasing evidence of cognitive decline in MSA [661, 662]; however, cognitive deficits are poorly recognized in this condition and in fact are listed as nonsupportive diagnostic features in the latest consensus diagnostic criteria [656] for MSA. In the later stages of the illness, a variety of respiratory and sleep disturbances add to the progressive disability. Occasionally, respiratory dysfunction, particularly dysrhythmic breathing in wakefulness that becomes worse during sleep, manifests in the initial stage of the illness [663]. In the final stage, progressive autonomic and somatic dysfunctions are compounded by respiratory failure. Ventilatory disturbances now may be present in both wakefulness and sleep. Pathologically, there are various combinations of striatonigral degeneration, OPCA, and degeneration of the autonomic neurons. A distinctive neuropathologic alteration in MSA is thought to be the presence of argyrophilic oligodendroglial cytoplasmic inclusions in the cortical motor, premotor, and supplementary motor areas; extrapyramidal and corticocerebellar systems; brain stem reticular formation; and supraspinal autonomic systems and their targets [653]. This inclusion-bearing oligodendroglial degeneration may cause or contribute to the manifestations of clinical symptoms in MSA. In fact, Wenning et al. [654] recently hypothesized that MSA is a primary oligodendrogliopathy, followed by the secondary selective neurodegeneration. In fact, a prion-like transfer of α-synuclein from neurons to oligodendrocytes has been suggested to be playing a crucial role in the pathogenesis and progression of MSA [659].
Sleep Disturbances
Patients with autonomic failure (primary or secondary) often have sleep dysfunction. There is a bidirectional relationship between disorders of ANS and sleep [644, 664, 665]: Sleep disorders may affect ANS functions, and ANS disorders may affect physiology of sleep. Because of this close associated, every patient presenting with AF should be questioned about sleep dysfunction; similarly, one should be vigilant about a possible autonomic impairment in a primary sleep disorder and look for clues to AF.
Sleep dysfunction is very common in MSA and includes insomnia with sleep fragmentation; EDS and RBD, which may occasionally be the presenting feature [666, 667]; and sleep-related respiratory dysrhythmias. RBD is very common, being present in 80–95 % of MSA patients [200, 664–677]. The characteristic clinical features of RBD include intermittent loss of REM-related muscle atonia and appearance of a variety of abnormal motor activities during sleep. The patient presents a violent dream-enacting behavior during REM sleep, often causing self-injury or injury to the bed partner. RBD may precede the illness or may present concomitantly or after the onset of MSA [664–677]. Positron-emission tomography (PET) and single-photon-emission computed tomography (SPECT) studies by Gilman et al. [671] suggested that RBD in MSA is related to nigrostriatal dopaminergic deficit. In other cases, RBD in MSA may be due to the neuropathologic changes in the brain stem REM-generating neurons.
The most common sleep disorders in MSA result from a variety of sleep-related respiratory dysrhythmias similar to those described in other neurologic conditions (see Fig. 41.9). The most common types of respiratory dysrhythmias consist of sleep apnea and hypopnea associated with repeated arousals and hypoxemia [85, 87, 91, 663, 664, 671, 678, 692], dysrhythmic breathing [87, 91, 663], and laryngeal stridor due to laryngeal abductor paralysis [647, 648, 684, 689–692]. Less commonly, apneustic breathing [679] and inspiratory gasping [85, 90, 647, 684] may occur. Hypersomnia often results from nocturnal sleep disruption. Sudden nocturnal death in patients with MSA in some cases probably is due to respiratory arrest and other cardiorespiratory abnormalities. Sleep-related respiratory dysrhythmias in MSA are present in almost 100 % of the cases in the advanced stages of the illness. Polysomnographic studies may show the following: a reduction of total sleep time, decreased sleep efficiency, increased number of awakenings during sleep, a reduction of slow-wave and REM sleep, absence of muscle atonia in REM sleep in those with RBD, and a variety of respiratory dysrhythmias. Laryngeal stridor and excessive snoring resulting from laryngeal abductor paralysis have been described in cases of MSA by several groups [648, 680, 682–684, 689–692]. The nocturnal stridor can be inspiratory, expiratory, or both and can cause upper airway obstruction during sleep. The stridor may result in a striking noise likened to a donkey’s braying [87, 648]. Williams’s group [682] noted this abnormality in 8 of 12 cases. The stridor can be relieved by tracheostomy. The group led by Guilleminault [685–687] described eight patients with predominantly upper airway OSA associated with O2 desaturation.
In our early study of four patients with MSA [99], we observed periodic central apnea in the erect position (Fig. 41.12) and CSB in one patient during the last stage of the illness. Impaired hypercapnic ventilatory response and mouth occlusion pressure response in the supine position in one patient suggested impairment of the metabolic respiratory system, whereas normal hypercapnic and hypoxic ventilatory responses in another patient (in the presence of an abnormal respiratory pattern resembling that noted by Lockwood [678]) suggested that the chemoreceptor control and respiratory pattern generator were probably subserved by different populations of neurons rendered selectively vulnerable in MSA. The neuropathologic findings in the same patient with impaired chemoreceptor response—neuronal loss and astrocytosis in the pontine tegmentum—suggested involvement of the respiratory neurons in the brain stem. In a later study [85, 87], we described 10 other patients with MSA who showed central apnea, including CSB or Cheyne–Stokes variant type breathing and upper airway obstructive and mixed apneas accompanied by O2 desaturation, predominantly during NREM sleep stages 1 and 2 and REM sleep (Fig. 41.26). During sleep, seven patients had central apnea, two had upper airway OSA, and three had mixed apneas. The AHI varied from 20 to 80; the duration of apneas ranged from 10 to 65 s. The variation in the heart rate during apneic and eupneic cycles was not seen in these patients with the evidence of cardiac autonomic denervation. This finding was in contrast to the bradyarrhythmias and tachyarrhythmias noted during apnea and immediately after resumption of normal breathing in patients with primary sleep apnea syndrome [88]. Four patients had several episodes of central apneas during relaxed wakefulness; it was as if the respiratory center “forgot” to breathe. Two patients had inspiratory gasps and two required tracheostomy for respiratory dysrhythmia. All-night PSG studies in two patients revealed the following sleep abnormalities in addition to recurrent episodes of sleep apneas accompanied by O2 desaturation: marked reduction of NREM sleep stages 3 and 4 and REM sleep, increased awakenings after sleep onset, snoring, and excessive body movements and frequent arousal responses in the EEG. In 8 of 10 patients, dysrhythmic breathing occurred mostly during sleep, although in 4 of these 8 it was also present during wakefulness; this finding suggests that this type of respiratory dysrhythmia is very common in MSA. These observations are in agreement with the suggestion of McNicholas et al. [663] that such findings imply an impaired respiratory pattern generator in these patients.


Fig. 41.26
A portion of an episode of mixed apnea during stage 2 NREM sleep associated with oxygen desaturation in a patient with multiple system atrophy. The electroencephalogram (EEG) is shown in the top six channels. Also shown are electromyography (EMG) of the mentalis (MENT) and intercostal (INT) muscles, electrocardiogram (EKG), nasal and oral airflow, and abdominal pneumogram (ABD PNEUMO). Reproduced with the permission from Chokroverty [91]
Mechanisms of Ventilatory Dysrhythmia
There is ample evidence in the literature [85, 87, 108, 646–648, 653–655, 657, 659, 660] of pathologic involvement of the pontine tegmentum, reticular formation, NTS, nucleus ambiguus, hypoglossal nucleus, and, in some patients, anterior horn cells of the cervical and thoracic spinal cord. Lockwood [678] and Chokroverty et al. [99] correlated the physiologic and clinical findings of respiratory dysrhythmias with a direct involvement of the regions of the brain stem that contain the respiratory neurons. In addition, physiologic studies of respiratory control [99, 663] showing impairment of hypercapnic and hypoxic ventilatory and mouth occlusion pressure responses indirectly suggested an impairment of the metabolic respiratory control system. Vagal and sympathetic denervation in these patients is firmly established [327, 644, 646, 659, 660]. The pathogenic mechanisms for the respiratory dysrhythmia include all that had been postulated in the beginning of this chapter for the respiratory dysrhythmias in neurologic disorders. Additional mechanisms for the respiratory dysrhythmia in MSA have been suggested [85]: interference with the forebrain, midbrain, and pontine inputs to the medullary respiratory neurons causing dysrhythmic and apneustic breathing; involvement of the direct projections from the hypothalamus and central nucleus of the amygdala to the respiratory neurons in the NTS and nucleus ambiguus; affection (loss of neurons) [644, 693] of the parabrachial nucleus (PBN) and the adjacent Kölliker–Fuse nucleus (KFN) in the pons which are responsible for respiratory rhythmogenesis and control of upper airway resistance and have reciprocal connections with the medullary respiratory neurons including laryngeal motoneurons of the nucleus ambiguus; involvement of the vagal afferents from the lower and upper airway receptors, which would reduce the input to the central respiratory neurons, causing respiratory dysrhythmia; sympathetic denervation of the nasal mucosa causing increased nasal resistance, thus promoting upper airway obstructive apnea; and discrete neurochemical alterations that may interfere with the normal regulation of breathing.
There is experimental evidence that noradrenaline, serotonin, and dopamine play distinct roles in the control of breathing [644, 694]. Patients with MSA have been found to have low levels of dopamine and noradrenaline in the basal ganglia, the limbic-hypothalamic regions including the septal nuclei, and the LC [695]. Furthermore, these patients may also have specific catecholamine enzyme deficits in the brain and sympathetic ganglia [696]. SPECT findings by Gilman et al. [697] suggested decreased pontine cholinergic projections to the thalamus contributing to OSA in MSA. Finally, in a series of postmortem studies of brains obtained from patients with MSA, Benarroch and colleagues reported depletion of catecholaminergic neurons in the ventrolateral medulla [698], A5 noradrenergic neurons [699], cholinergic neurons in the medullary arcuate nucleus [700], corticotrophin-releasing factor neurons [701] in the putative pontine micturition center, mesopontine cholinergic neurons in the PPT and LDT nucleus [702, 703], ventrolateral medullary neurokinin 1 receptor-like immunoreactive neurons [704], chemosensitive glutamatergic and serotoninergic neurons in the arcuate nucleus in the ventral medullary surface as well as serotoninergic neurons in the medullary raphe [705], serotoninergic neurons in the pontomedullary raphe [706], and neurons in the ventrolateral nucleus ambiguus innervating the heart and dorsal vagal nucleus-innervating enteric neurons [707], loss of hypocretin (orexin) hypothalamic neurons [708], and loss of PBN and KFN in the pons [709]. Loss of these cell groups may contribute to the respiratory disturbances, including loss of automatic respiration and other autonomic dysfunction involving various systems in MSA [644, 693, 709].
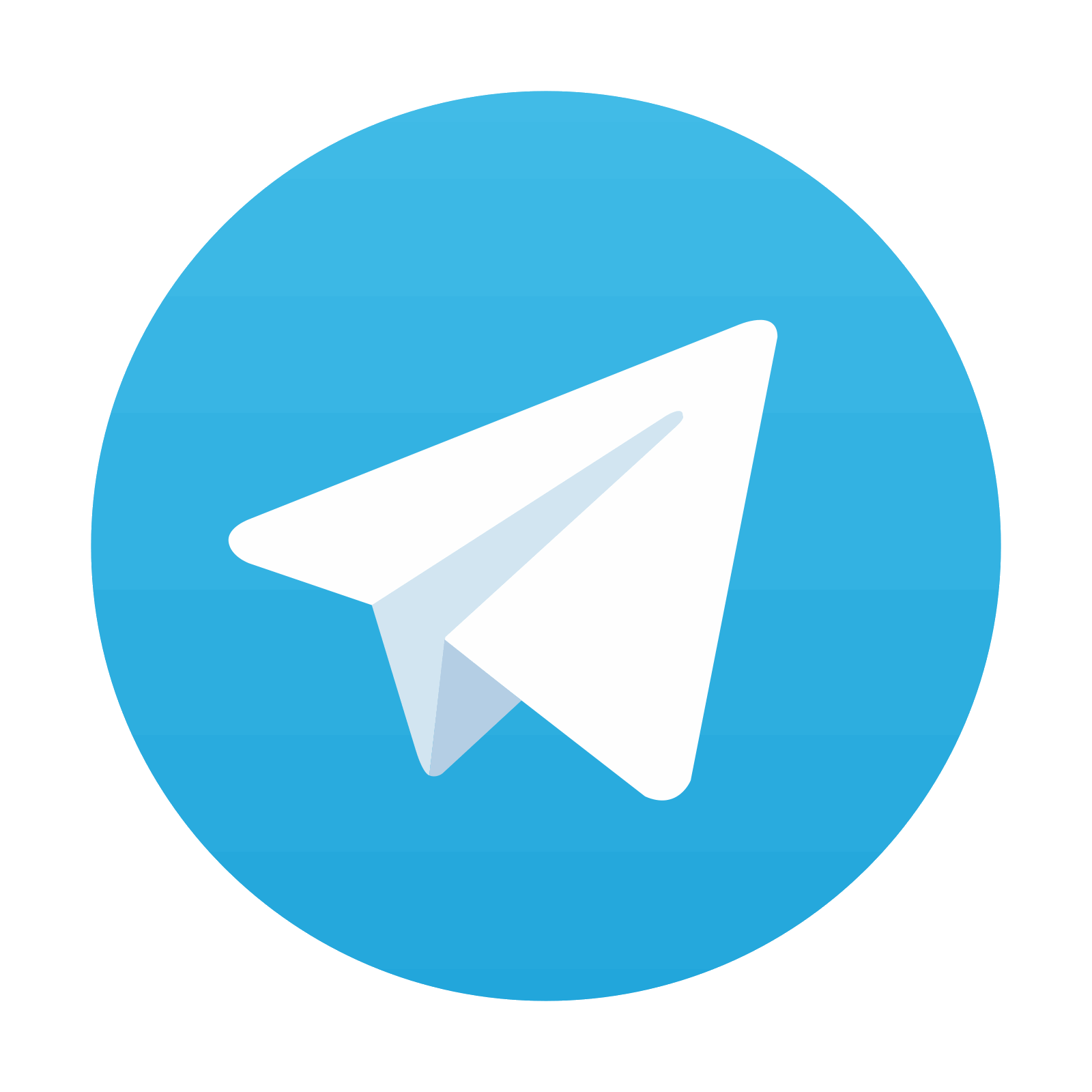
Stay updated, free articles. Join our Telegram channel
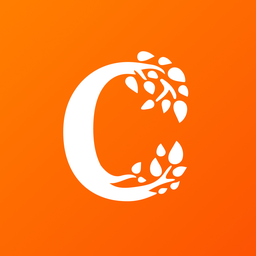
Full access? Get Clinical Tree
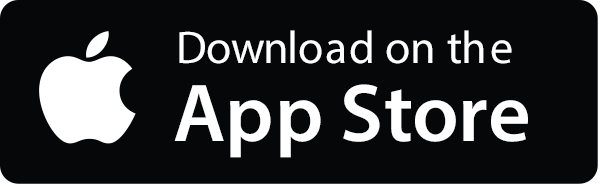
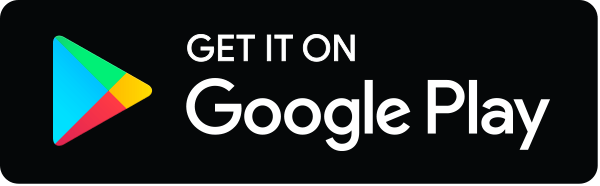