Spinal Reflexes
Reflexes Are Adaptable to Particular Motor Tasks
Spinal Reflexes Produce Coordinated Patterns of Muscle Contraction
Cutaneous Reflexes Produce Complex Movements That Serve Protective and Postural Functions
Local Spinal Circuits Contribute to the Coordination of Reflex Responses
Ia Inhibitory Interneurons Coordinate the Muscles Surrounding a Joint
Divergence in Reflex Pathways Amplifies Sensory Inputs and Coordinates Muscle Contractions
Convergence of Inputs on Ib Interneurons Increases the Flexibility of Reflex Responses
Central Neurons Can Regulate the Strength of Spinal Reflexes at Three Sites in the Reflex Pathway
Gamma Motor Neurons Adjust the Sensitivity of Muscle Spindles
Proprioceptive Reflexes Play an Important Role in Regulating Both Voluntary and Automatic Movements
Reflexes Involving Limb Muscles Are Mediated Through Spinal and Supraspinal Pathways
Interruption of Descending Pathways to the Spinal Cord Frequently Produces Spasticity
Transection of the Spinal Cord in Humans Leads to a Period of Spinal Shock Followed by Hyperreflexia
DURING PURPOSEFUL MOVEMENTS the central nervous system uses information from a vast array of sensory receptors to ensure that the pattern of muscle activity suits the purpose. Without this sensory information movements tend to be imprecise, and tasks requiring fine coordination in the hands, such as buttoning one’s shirt, are impossible.
Charles Sherrington was among the first to recognize the importance of sensory information in regulating movements. In 1906 he proposed that simple reflexes—stereotyped movements elicited by activation of receptors in skin or muscle—are the basic units for movement. He further posited that complex sequences of movements can be produced by combining simple reflexes. This view guided motor physiology for much of the 20th century.
The view that reflexes are automatic, stereotyped movements in response to stimulation of peripheral receptors arose primarily from laboratory studies of reflexes in animals with central nervous system lesions. Once investigators began to measure reflexes in intact animals engaged in normal behavior, ideas about reflexes changed. We now know that reflexes are flexible, that under normal conditions they can be adapted to a task. The prevalent view today is that reflexes are integrated by centrally generated motor commands into complex adaptive movements.
In this chapter we consider the principles underlying the organization and function of reflexes, focusing on spinal reflexes. The sensory stimuli for spinal reflexes arise from receptors in muscles, joints, and skin, and the neural circuitry responsible for the motor response is entirely contained within the spinal cord.
Reflexes Are Adaptable to Particular Motor Tasks
A good example of the adaptability of reflexes is how certain reflexes change in response to stretching the wrist muscles. When a person is kneeling or standing the stretched muscles contract, but muscles in other limbs also contract to prevent a loss of balance. Interestingly, the reflex response of the elbow extensor of the opposite arm depends on how that arm is being used. If the arm is used to stabilize the body by holding the edge of a table, a large excitatory response in the elbow extensor muscle resists the forward sway of the body. If the arm is instead holding an unsteady object such as a cup of tea, reflex inhibition of the elbow extensors prevents movement of the cup (Figure 35-1A).
Figure 35-1 Reflex responses are often complex and can change depending on the task.
A. Perturbation of one arm causes an excitatory reflex response in the contralateral elbow extensor muscle when the contralateral limb is used to prevent the body from moving forward by grasping a table. The same stimulus produces an inhibitory response in the muscle when the contralateral hand holds a filled cup. (Adapted, with permission, from Marsden et al. 1981.)
B. Loading the thumb during a rhythmic sequence of finger-to-thumb pinching movements produces a reflex response in the finger muscle as well as the thumb muscle. The additional movement of the finger ensures that the pinching movement remains accurate. The blue area in the electromyogram (EMG) records indicates the reflex response. (Adapted, with permission, from Cole et al. 1984.)
Another example of adaptability is the reflex of finger and thumb flexor muscles in response to stretching thumb muscles. If a subject rhythmically taps the tips of the index finger and thumb to each other, and flexion of the thumb is resisted, a short-latency reflex response is produced in both the finger and thumb flexor muscles. As a result, the reflex in the finger flexor muscle produces a larger flexion movement of the finger to compensate for the reduced flexion of the thumb and ensure the performance of the intended task (Figure 35-1B). If the subject is simply making rhythmic thumb movements, a reflex response is produced only in the thumb flexor muscle.
A third example of adaptability is the conditioning of the flexion-withdrawal reflex. Flexion-withdrawal can be associated with an auditory tone by classical conditioning (see Chapter 65). A subject is asked to place the palmar surface of an index finger on an electrode. A mild electrical shock is then paired with an audible tone. As expected, after only a few pairings the tone alone will elicit the withdrawal reflex. What exactly has been conditioned? Is it the contraction of a fixed group of muscles or the behavioral act that withdraws the finger from the noxious stimulus?
This question can be answered by having the subject turn his or her hands over after conditioning is complete, so that now the dorsal surface of the finger is in contact with the electrode. Most subjects will withdraw their fingers from the electrode when the tone is played, even though this means that the opposite muscles now contract. Thus, the conditioned response is not merely a stereotyped set of muscle contractions but the elicitation of an appropriate behavior.
Three important principles are illustrated by these examples. First, neural signaling in reflex pathways is adjusted according to the motor task. The state of the reflex pathways for any task is referred to as the functional set. Exactly how a functional set is established for most motor tasks is largely unknown, and unraveling the underlying mechanisms is one of the challenging areas of contemporary research on motor systems. Second, sensory input from a localized source generally produces coordinated reflex responses in several muscles at once, some of which may be distant from the stimulus. Third, supraspinal centers play an important role in modulating and adapting spinal reflexes, even to the extent of reversing movements when appropriate.
To understand the neural basis for reflexes and how they are modified for a particular task, we must first have a thorough knowledge of how reflex pathways are organized in the spinal cord. Although under normal conditions descending central commands directly shape spinal reflexes, many qualitative features of spinal reflexes are maintained after complete transection of the spinal cord, a condition that isolates the spinal circuits from the brain.
Spinal Reflexes Produce Coordinated Patterns of Muscle Contraction
Cutaneous Reflexes Produce Complex Movements That Serve Protective and Postural Functions
A familiar example of a spinal reflex is the flexion-withdrawal reflex, in which a limb is quickly withdrawn from a painful stimulus. Flexion-withdrawal is a protective reflex in which a discrete stimulus causes all the flexor muscles in that limb to contract coordinately. We know that this is a spinal reflex because it persists after complete transection of the spinal cord.
The sensory signal activates divergent polysynaptic reflex pathways. One excites motor neurons that innervate flexor muscles of the stimulated limb, whereas another inhibits motor neurons that innervate the limb’s extensor muscles (Figure 35-2A). Excitation of one group of muscles and inhibition of their antagonists—those that act in the opposite direction—is what Sherrington called reciprocal innervation, a key principle of motor organization that is discussed later in this chapter.
Figure 35-2 Spinal reflexes involve coordinated contractions of numerous muscles in the limbs.
A. Polysynaptic pathways in the spinal cord mediate flexion and crossed-extension reflexes. One excitatory pathway activates motor neurons that innervate ipsilateral flexor muscles, which withdraw the limb from noxious stimuli. Another pathway simultaneously excites motor neurons that innervate contralateral extensor muscles, providing support during withdrawal of the limb. Inhibitory interneurons ensure that the motor neurons supplying antagonist muscles are inactive during the reflex response. (Adapted, with permission, from Schmidt 1983.)
B. Monosynaptic pathways mediate stretch reflexes. Afferent axons from muscle spindles make excitatory connections on two sets of motor neurons: alpha motor neurons that innervate the same (homonymous) muscle from which they arise and motor neurons that innervate synergist muscles. They also act through interneurons to inhibit the motor neurons that innervate antagonist muscles. When a muscle is stretched by a tap with a reflex hammer, the firing rate in the afferent fiber from the spindle increases. This leads to contraction of the same muscle and its synergists and relaxation of the antagonist. The reflex therefore tends to counteract the stretch, enhancing the spring-like properties of the muscles. The records on the right demonstrate the reflex nature of contractions produced by muscle stretch in a decerebrate cat. When an extensor muscle is stretched it normally produces a large force, but it produces a very small force (dashed line) after the sensory afferents in the dorsal roots have been severed. (Adapted, with permission, from Liddell and Sherrington 1924.)
The reflex can produce an opposite effect in the contralateral limb, that is, excitation of extensor motor neurons and inhibition of flexor motor neurons. This crossed-extension reflex serves to enhance postural support during withdrawal of a foot from a painful stimulus. Activation of the extensor muscles in the opposite leg counteracts the increased load caused by lifting the stimulated limb. Thus, flexion-withdrawal is a complete, albeit simple, motor act.
Although flexion reflexes are relatively stereotyped, both the spatial extent and the force of muscle contraction depend on stimulus intensity. Touching a stove that is slightly hot may produce moderately fast withdrawal only at the wrist and elbow, whereas touching a very hot stove invariably leads to a forceful contraction at all joints, leading to a rapid withdrawal of the entire limb. The duration of the reflex usually increases with stimulus intensity, and the contractions produced in a flexion reflex always outlast the stimulus.
Because of the similarity of the flexion-withdrawal reflex to stepping, it was once thought that the flexion reflex is important in producing contractions of flexor muscles during walking. We now know, however, that a major component of the neural control system for walking is a set of intrinsic spinal circuits that do not require sensory stimuli (see Chapter 36). Nevertheless, in mammals the intrinsic spinal circuits that control walking share many of the interneurons that are involved in flexion reflexes.
The Stretch Reflex Resists the Lengthening of a Muscle
Perhaps the most important—certainly the most studied—spinal reflex is the stretch reflex, a lengthening contraction of a muscle. Stretch reflexes were originally thought to be an intrinsic property of muscles. But early in the last century Liddell and Sherrington showed that they could be abolished by cutting either the dorsal or the ventral root, thus establishing that these reflexes require sensory input from muscle to spinal cord and a return path to muscle (Figure 35-2B).
We now know that the receptor that senses the change of length is the muscle spindle (Box 35-1) and that the type Ia axon from this receptor makes direct excitatory connections with motor neurons. (The classification and nomenclature of sensory fibers from muscle are discussed in Box 35-2.) The afferent axon also connects to interneurons that inhibit the motor neurons that innervate antagonist muscles, another instance of reciprocal innervation. This inhibition prevents muscle contractions that might otherwise resist the movements produced by the stretch reflexes.
Muscle spindles are small encapsulated sensory receptors that have a spindle-like or fusiform shape and are located within the fleshy part of a muscle. Their main function is to signal changes in the length of the muscle within which they reside. Changes in length of muscles are closely associated with changes in the angles of the joints that the muscles cross. Thus muscle spindles are used by the central nervous system to sense relative positions of the body segments.
Each spindle has three main components: (1) a group of specialized intrafusal muscle fibers with central regions that are noncontractile; (2) sensory fibers that terminate in the noncontractile central regions of the intrafusal fibers; and (3) motor axons that terminate in the polar contractile regions of the intrafusal fibers (Figure 35-3A).
Figure 35-3 The muscle spindle detects changes in muscle length.
A. The main components of the muscle spindle are intrafusal muscle fibers, afferent sensory endings, and efferent motor endings. The intrafusal fibers are specialized muscle fibers with central regions that are not contractile. Gamma motor neurons innervate the contractile polar regions of the intrafusal fibers. Contraction of the polar regions pulls on the central regions of the intrafusal fiber from both ends. The sensory endings spiral around the central regions of the intrafusal fibers and are responsive to stretch of these fibers. (Adapted, with permission, from Hulliger 1984.)
B. The muscle spindle contains three types of intrafusal fibers: dynamic nuclear bag, static nuclear bag, and nuclear chain fibers. A single Ia sensory axon innervates all three types of fibers, forming a primary sensory ending. Type II sensory axons innervate the nuclear chain fibers and static bag fibers, forming a secondary sensory ending. Two types of motor neurons innervate different intrafusal fibers. Dynamic gamma motor neurons innervate only dynamic bag fibers; static gamma motor neurons innervate various combinations of chain and static bag fibers. (Adapted, with permission, from Boyd 1980.)
C. Selective stimulation of the two types of gamma motor neurons has different effects on the firing of the Ia fibers from the spindle. Without gamma stimulation the Ia fiber shows a small dynamic response to muscle stretch and a modest increase in steady-state firing. When a static gamma motor neuron is stimulated, the steady-state response of the Ia fiber increases, but there is a decrease in the dynamic response. When a dynamic gamma motor neuron is stimulated, the dynamic response of the Ia fiber is markedly enhanced, but the steady-state response gradually returns to its original level. (Adapted, with permission, from Brown and Matthews 1966.)
When the intrafusal fibers are stretched, often referred to as “loading the spindle,” the sensory nerve endings are also stretched and increase their firing rate. Because muscle spindles are arranged in parallel with the extrafusal muscle fibers that make up the main body of the muscle, the intrafusal fibers change in length as the whole muscle changes. Thus, when a muscle is stretched, activity in the sensory endings of muscle spindles increases. When a muscle shortens, the spindle is unloaded and the activity decreases.
The intrafusal muscle fibers are innervated by gamma motor neurons, which have small-diameter myelinated axons, whereas the extrafusal muscle fibers are innervated by alpha motor neurons, with large-diameter myelinated axons. Activation of gamma motor neurons causes shortening of the polar regions of the intrafusal fibers. This in turn stretches the central region from both ends, leading to an increase in firing rate of the sensory endings or to a greater likelihood that the sensory endings will fire in response to stretch of the muscle. Thus the gamma motor neurons adjust the sensitivity of the muscle spindles. Contraction of the intrafusal muscle fibers does not contribute significantly to the force of muscle contraction.
The structure and functional behavior of muscle spindles is considerably more complex than this simple description implies. When a muscle is stretched the change in length has two phases: a dynamic phase, the period during which length is changing, and a static or steady-state phase, when the muscle has stabilized at a new length. Structural specializations within each component of the muscle spindles allow spindle afferents to signal aspects of each phase separately.
There are two types of intrafusal muscle fibers: nuclear bag fibers and nuclear chain fibers. The bag fibers can be divided into two groups, dynamic and static. A typical spindle has two or three bag fibers and a variable number of chain fibers, usually about five. Furthermore, the intrafusal fibers receive two types of sensory endings. A single Ia (large diameter) axon spirals around the central region of all intrafusal muscle fibers and serves as the primary sensory ending (Figure 35-3B). A variable number of type II (medium diameter) axons, located adjacent to the central regions of the static bag and chain fibers, serve as secondary sensory endings.
The gamma motor neurons can also be divided into two classes: Dynamic gamma motor neurons innervate the dynamic bag fibers, whereas the static gamma motor neurons innervate the static bag fibers and the chain fibers.
This duality of structure is reflected in a duality of function. The tonic discharge of both primary and secondary sensory endings signals the steady-state length of the muscle. The primary sensory endings are, in addition, highly sensitive to the velocity of stretch, allowing them to provide information about the speed of movements. Because they are highly sensitive to small changes, the primary endings rapidly provide information about sudden unexpected changes in length, which can be used to generate quick corrective reactions.
Increases in the firing rate of dynamic gamma motor neurons increase the dynamic sensitivity of primary sensory endings but have no influence on secondary sensory endings. Increases in the firing rate of static gamma motor neurons increase the tonic level of activity in both primary and secondary sensory endings, decrease the dynamic sensitivity of primary endings (Figure 35-3C), and can prevent the silencing of primary endings when a muscle is released from stretch. Thus the central nervous system can independently adjust the dynamic and static sensitivity of the different sensory endings in muscle spindles.
Sherrington developed an experimental model for investigating spinal circuitry that is especially valuable in the study of stretch reflexes. He conducted his experiments on cats whose brain stems had been surgically transected at the level of the midbrain, between the superior and inferior colliculi. This is referred to as a decerebrate preparation. The effect of this procedure is to disconnect the rest of the brain from the spinal cord, thus blocking sensations of pain as well as interrupting normal modulation of reflexes by higher brain centers. A decerebrate animal has stereotyped and usually heightened stretch reflexes, making it is easier to examine the factors controlling their expression.
Sensory fibers are classified according to their diameter. Axons with larger diameters conduct action potentials more rapidly than do fibers of smaller diameters. Because each class of sensory receptors is innervated by fibers with diameters within a restricted range, this method of classification distinguishes to some extent the fibers that arise from the different types of receptor organs. The main groups of sensory fibers from muscle are listed in Table 35-1.
The organization of reflex pathways in the spinal cord has been established primarily by electrically stimulating the sensory fibers and recording evoked responses in different classes of neurons in the spinal cord. This method of activation has three advantages over natural stimulation. The timing of afferent input can be precisely established; the responses evoked in motor neurons and other neurons by different classes of sensory fibers can be assessed by grading the strength of the electrical stimulus; and certain classes of receptors can be selectively activated.
The strength of the electrical stimulus required to activate a sensory fiber is measured relative to the strength required to activate the afferent fibers with the largest diameter because these fibers have the lowest threshold for electrical activation. The threshold of type I fibers is usually one to two times that of the largest afferents (with Ia fibers having, on average, a slightly lower threshold than Ib fibers). For most type II fibers the threshold is 2 to 5 times higher, whereas type III and IV have thresholds in the range of 10 to 50 times that of the largest afferents.
Without control by higher brain centers, descending pathways from the brain stem powerfully facilitate the neuronal circuits involved in the stretch reflexes of extensor muscles. This results in a dramatic increase in extensor muscle tone that sometimes suffices to support the animal in a standing position. In normal animals, owing to the balance between facilitation and inhibition, stretch reflexes are weaker and considerably more variable in strength than those in decerebrate animals.
Local Spinal Circuits Contribute to the Coordination of Reflex Responses
The Stretch Reflex Involves a Monosynaptic Pathway
The neural circuit responsible for the stretch reflex was one of the first reflex pathways to be examined in detail. The physiological basis of this reflex was examined by measuring the latency of the response in ventral roots to electrical stimulation of dorsal roots. When the Ia sensory axons innervating the muscle spindles were selectively activated, the reflex latency through the spinal cord was less than 1 ms. This demonstrated that the Ia fibers make direct connections on the alpha motor neurons, for the delay at a single synapse is typically 0.5 ms to 0.9 ms (Figure 35-4B).
Figure 35-4 The number of synapses in a reflex pathway can be inferred from intracellular recordings.
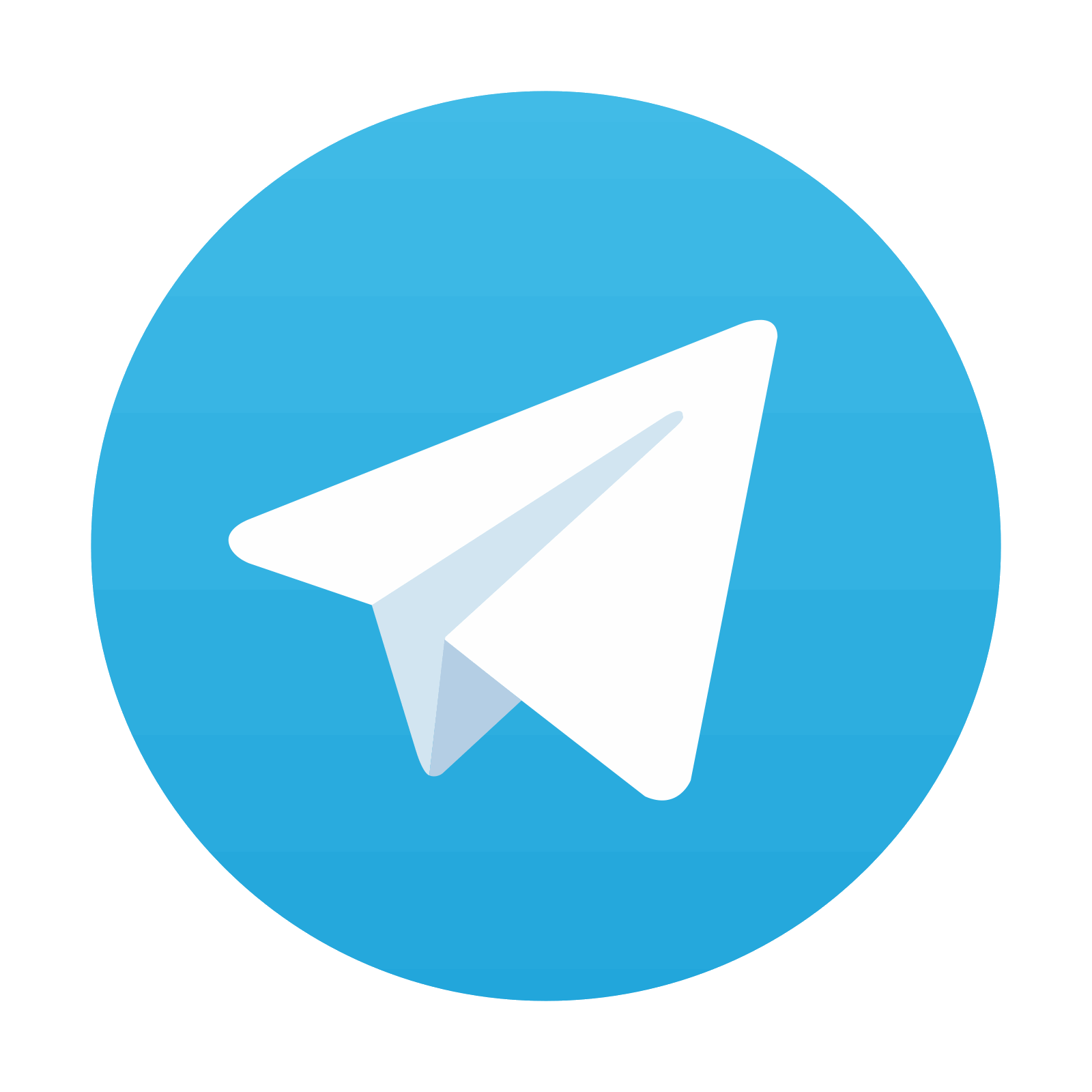
Stay updated, free articles. Join our Telegram channel
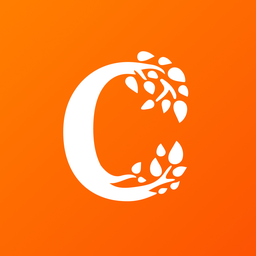
Full access? Get Clinical Tree
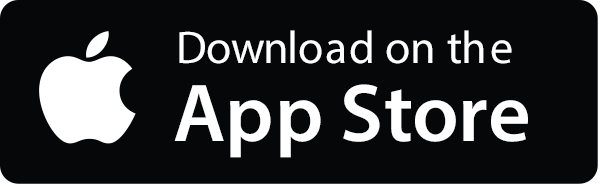
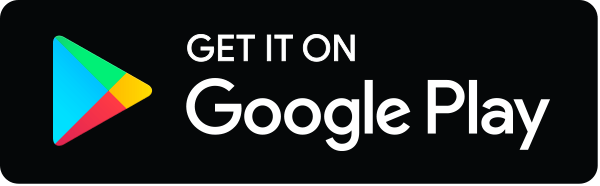