Fig. 1.1
(I) Initial observation that repeated electroconvulsive shocks induce self-sustaining SE in rats. Representative electrographic recordings from skull screw electrodes in paralyzed and O2-ventilated rats maintained in good acid-base balance. Animals shocked repeatedly for 25 min (50 ECS) or longer showed self-sustaining seizure activity after the end of electrical stimulation. Increasing the duration of stimulation resulted in longer lasting self-sustaining SE (Reproduced from [14], @ Elsevier 1972 and 15 @ Epilepsia). (II) EEG during SE induced by 30 min of intermittent perforant path stimulation (PPS). (a) Representative course of spikes. (b) 24 h distribution of seizures (black bars). The period of stimulation is indicated by the gray bar on the top. Each line represents 2 h of monitoring. (c) Evolution of EEG activity in the dentate gyrus during SE. Software- recognized seizures are underlined (Reproduced with changes from [31], © Elsevier, 1998). (III) The effects of NMDA (a–d) and AMPA/kainate (e) receptor blockers on SE induced by PPS. Each graph shows frequency of spikes plotted against time during SE. PPS is indicated by the gray bar. Representative time course of seizures detected by the software is shown in the graphs immediately to the right. Each line represents 2 h of monitoring, and each seizure is indicated by a black bar. Arrows indicate drug administration. Notice that in this model, NMDA receptor blockers MK-801 (0.5 mg/kg i.p.), 2,5-DCK (10 nmol into the stimulated hilus), and ketamine (10 mg/kg i.p.) are administered 10 min after the end of PPS aborted SE soon after injection. CNQX (10 nmol into the hilus) injected after PPS induced only transient suppression of seizures, which reappeared 2–4 h after CNQX injection (Reproduced with changes from [72], © Elsevier 1999). (IV) Time-dependent development of pharmacoresistance in SE induced by 60 min PPS. (a) Pretreatment with diazepam (DZP) or phenytoin (PHT) prevented the development of SE. (b) Top: When injected 10 min after PPS, neither of them aborted SSSE, although they shortened its duration. *p < 0.05 vs. control. #p < 0.05 vs. DZP and PHT, respectively, in a (pretreatment). Open bars show cumulative seizure time, and black bars show the duration of SE. (c–e) Representative time course of seizures in a control animal (c), an animal pretreated with diazepam (d), or an animal injected with diazepam 10 min after PPS (e). Each line represents 2 h of EEG monitoring. Each software-recognized seizure is shown by a small black bar. PPS is indicated by gray bars on the top. Injection of diazepam is indicated by an arrow in d and e. Notice that, in the control animal, SE lasted for 17 h. In diazepam-pretreated rats, seizures occurred during PPS, but only a few seizures were observed within the first 20 min after PPS. In the diazepam-posttreated animal , SE continued for 8 h. (Reproduced with changes from 44 , © Elsevier 1998)
Following the discovery of the kindling phenomenon, Taber et al. [16] and de Campos and Cavalheiro [17] modified the method of stimulation to obtain SE. McIntyre et al. [18, 19] showed that continuous stimulation for 60 min of basolateral amygdala of kindled animals induced SE, demonstrating that the kindled state predisposes to the development of SE. Both high-[20] and low-frequency [21] stimulation of limbic structures can induce SSSE. Inoue et al. [22, 23] produced SE in naïve rats by electrical stimulation of prepiriform cortex. Handforth and Ackerman [24, 25] used continuous high-frequency stimulation of the hippocampus or amygdala and analyzed the functional anatomy of SE with [14C]-deoxyglucose. They delineated several types of SE, ranging from a very restricted limbic pattern around the site of stimulation with mild behavioral manifestations to bilateral involvement of limbic and extralimbic structures accompanied by widespread clonic seizures. Lothman and colleagues [26] showed that stimulation of dorsal hippocampus for 60 min with high-frequency trains with very short inter-train intervals, a protocol which they called “continuous hippocampal stimulation” (CHS), resulted in the development, in many animals, of SE characterized by non-convulsive or mild convulsive seizures which lasted for hours after the end of CHS . Metabolic activity was increased in many brain structures and decreased in others [27]; these seizures lead to loss of GABAergic hippocampal inhibition, to hippocampal interictal spiking, and to delayed (1 month after CHS) spontaneous seizures [28].
Vicedomini and Nadler [29] showed that intermittent stimulation of excitatory pathways could generate SE in many regions. SE developed in each animal that showed at least ten consecutive afterdischarges. We used a protocol derived from those of Vicedomini and Nadler [29] and Sloviter [30]. We stimulated the perforant path in awake rats with 10 s, with 20 Hz trains (1 ms square wave, 20 V) delivered every minute, and with 2 Hz continuous stimulation and recorded from dentate gyrus [31] (Fig. 1.1-II). Nissinen et al. [32] developed a similar model based on amygdala stimulation. Other variations of the perforant path model have been used [33]. The perforant path stimulation model provides a tool to study epileptic pathways, histopathological changes, sequelae of SE, systemic factors, as well as genetic background influencing the physiology of SE and to test the effects of AEDs. The EEG and clinical evolution of SE (Fig. 1.1 -I) is similar to that described for clinical SE [34, 35], starting with individual seizures which merge into nearly continuous polyspikes, which later are interrupted by slow waves, which increase in duration and interrupt seizure activity while polyspikes decrease in amplitude. Eventually, after many hours, this evolves into a burst-suppression pattern of progressively decreasing power.
Chemical Models of SE: Pilocarpine and Lithium–Pilocarpine
Pilocarpine is an agonist at muscarinic receptors. Its induction of SE has been shown to occur primarily through activation of muscarinic 1 receptors (M1R), but its actions on muscarinic 2 receptors (M2R) may also contribute to the SE propagation and sequelae development by affecting systemic factors, such as inflammation [36]. Lithium is administered prior to pilocarpine in order to decrease animal mortality, but pilocarpine may be used alone.
Many different protocols of pilocarpine administration exist [37]. Pilocarpine is injected either systemically (i.e., intraperitoneal route) or directly into the brain (intracerebroventricular or intrahippocampal route), while electrical activity is being monitored in the cortex and hippocampus. The amount of injected chemical can be variable, depending on intended outcome. Male rats or mice are most often used in these experiments, but females have shown similar responses. Just as with electrical stimulation models, and similar to human patients, induction of SE with pilocarpine leads to increased synaptic activity in limbic areas. Acutely, following injections of pilocarpine, normal hippocampal and cortical rhythms are transformed to spiking and then electrographic seizures, which within an hour after injection become sustained, similar to human SE . This electrical activity is correlated with behavior, which consists of facial automatisms, akinesia, ataxia, and eventually motor seizures and SE. Pathologic changes seen following induction of SE are similar to those seen in human brains and are an important paradigm in our effort to understand the pathologic process of epileptogenesis.
Turski et al. [9] developed the pilocarpine model of SE. Honchar and Olney [11] showed that lithium pretreatment reduces the dose of pilocarpine needed and the mortality from SE. Buterbaugh [38] and Morrisett et al. [39] showed that, in these chemical models, seizures become independent from the initial trigger, and self-sustaining, as they do in electrical stimulation models. Morissett et al. [39] administered atropine sulfate, which removed the cholinergic stimulus. This was effective in blocking status epilepticus when given before the onset of behavioral seizures, but failed to stop SE after onset of overt seizures, demonstrating that different mechanisms are responsible for initiation and maintenance of SE and that self-sustaining SE can be triggered by chemical as well as electrical stimulation. These results were extended to juvenile animals by Suchomelova et al. [40]. The pilocarpine model can also be used to test potential AEDs for their effects on SE, as well as on the induction and evolution to chronic epilepsy.
Studies of the Transition from Single Seizures to SE: GABAAR
GABAergic agents lose their therapeutic effectiveness as status epilepticus (SE) proceeds, and brief convulsant stimuli result in a diminished inhibitory tone of hippocampal circuits [41], as indicated by loss of paired-pulse inhibition in vivo. To examine the effects of SE on GABAA synapses, whole-cell patch-clamp recordings of GABAA miniature inhibitory postsynaptic currents (mIPSCs) were obtained from dentate gyrus granule cell in hippocampal slices from 4- to 8-week-old Wistar rats after 1 h of lithium–pilocarpine SE and compared to controls [42]. Figure 1.2a shows that mIPSCs recorded from granule cells in slices prepared 1 h into SE showed a decreased peak amplitude to 61.8 ± 11.9% of controls (−31.5 ± 6.1 picoAmpere (pA) for SE vs. –51.0 ± 17.0 pA for controls; p < 0.001) and an increase of decay time to 127.9 ± 27.6% of controls (7.75 ± 1.67 ms for SE vs. 6.06 ± 1.17 ms for controls; p < 0.001). Unlike mIPSCs, tonic currents (Fig. 1.2b) increased in amplitude to a mean of −130.0 (±73.6) pA in SE vs. −44.8(±19.2) pA in controls (p < 0.05). Tonic currents are thought to be mediated by extrasynaptic receptors containing δ subunits, which are known to display low levels of desensitization and internalization. Their persistence during SE might suggest that drugs with strong affinity for extrasynaptic receptors, such as neurosteroids, may be effective. Mathematical modeling of GABAA synapses using mean–variance fluctuation analysis and seven-state GABAA receptor models suggested that SE reduced postsynaptic receptor number by 47% [from 38 ± 15 (control) to 20 ± 6 (SE) receptors per synapse; p < 0.001] (Fig. 1.2a). This may underestimate the acute changes, since slices collected from animals in SE were examined after 1–2 seizure-free hours in vitro.


Fig. 1.2
(a) γ-Aminobutyric acid (GABA)A miniature inhibitory postsynaptic currents (IPSCs) recorded from the soma of granule cells in hippocampal slices prepared from rats in lithium–pilocarpine-induced SE for 1 h show reduced amplitude but little change in kinetics. Our model predicts that this reflects reduced number of GABAA receptors from 38 ± 15 in controls to 20 ± 6 per synapse in slices from animals in SE. (b) In slices from rats in SE, tonic currents generated by extrasynaptic GABAA receptors are increased, reflecting (at least in part) increased extracellular GABA concentration during SE. (c) Subcellular distribution of β2–3 subunits of GABAA receptors after 1 h of SE. In control granule cells (left) the β2–3 subunits of GABAA receptors (red) localize to the vicinity of the presynaptic marker synaptophysin (green), whereas after an hour of SE induced by lithium and pilocarpine (right), many have moved to the cell interior. (d) The graph shows an increase in β2–3 subunits internalization following SE in the hilus and in the dentate gyrus granule cell layer. (e) NMDA miniature excitatory postsynaptic currents (NMDA-mEPSCs) mean traces from a typical granule cell from a control (red) and a SE animal (black), demonstrating larger amplitude and area-under-the curve (AUC) in the latter, indicating an increased response of the postsynaptic membrane to a quantum of glutamate released from a single vesicle, and suggesting an increase in NMDAR from 5 ± 1 NMDAR/synapse in controls to 8 ± 2 NMDAR/synapse in slices from rats in SE. (f) Subcellular distribution of NMDA NR1 subunit-like immunoreactivity (LI) after 1 h of SE. Hippocampal sections through CA3 of control (a1) and SE (b1) brains stained with antibodies against the NR1 subunit-LI (red) and against the presynaptic marker synaptophysin-LI (green), with overlaps appearing yellow. Hippocampal sections of CA3 at higher magnification are shown in a2 and b2. Note increased NR1 subunit-LI colocalization with synaptophysin-LI in pyramidal cells for SE rat (bar—40 μm left panel; 10 μm right panel). (g) The number of colocalizations between NR1 subunits and synaptophysin increases with SE at both the soma and proximal dendrites of CA3 pyramidal cells (error bars as ± SEM). Modified from Naylor et al. ([42]: A-D. presented at a Meeting of Society of Neuroscience 2005) and 47: E-G, @ Elsevier 2013)
Immunocytochemistry was performed in rats perfused after 60 min of seizures induced by lithium–pilocarpine (Fig. 1.2c, d). These anatomical studies indicate that the decrease in number of synaptic receptors observed physiologically reflects, at least in part, receptor internalization. They show colocalization of the β2/3 subunits with the presynaptic marker synaptophysin on the surface of soma and proximal dendrites of dentate granule cells and CA3a pyramids in controls, with internalization of those subunits in SE (Fig. 1.2d). In the lithium–pilocarpine model at 60 min, 12 ± 17% of β2/3 subunits are internalized in control CA3 compared to 54 ± 15% in slices from rats in SE (p < 0.001). Numbers in CA1 were similar. We also found that the γ2 subunits are internalized during SE [42].
In conclusion, a decrease in synaptic GABAA currents and an increase in extrasynaptic tonic currents are observed with SE. Internalization/desensitization of postsynaptic GABAA receptors (possibly from increased GABA exposure) can explain the decreased amplitude of synaptic mIPSCs, although an increase in intracellular chloride concentration may also play a role. These changes at GABAergic synapses may represent important events in the transition from single seizures to self-sustaining SE (Fig. 1.1). Since internalized receptors are not functional, this internalization may reduce the response of inhibitory synapses to additional seizures and may in part explain the failure of inhibitory GABAergic mechanisms which characterizes the initiation phase of self-sustaining SE. The reduced synaptic receptor numbers also may explain the diminished effect of benzodiazepines and other GABAergic drugs as SE proceeds [43, 44] (Fig. 1.3, Table 1.1).


Fig. 1.3
Model summarizing our hypothesis on the role of receptor trafficking in the transition from single seizures to SE. After repeated seizures, the synaptic membrane surrounding GABAA receptors forms clathrin-coated pits (Cl), which internalize as clathrin-coated vesicles, inactivating the receptors since they are no longer within reach of the neurotransmitter GABA. These vesicles evolve into endosomes, which can deliver the receptors to lysosomes (L) where they are destroyed, or to the Golgi apparatus (G) from where they are recycled to the membrane. By contrast, in NMDA synapses, after repeated seizures, receptor subunits are mobilized to the synaptic membrane and assemble into additional receptors. As a result of this trafficking, the number of functional NMDA receptors per synapse increases while the number of functional GABAA receptors decreases [37, 41]. Reproduced from Chen and Wasterlain ([1] @ Elsevier 2006)
Table 1.1
Time-dependent changes in physiology of SE and treatment implications
Time | Changes/effects on excitability | Classes of medications working at this level and likely becoming inefficient due to status-induced changes in underlying physiology |
---|---|---|
Milliseconds–seconds | Protein phosphorylation Alternation in ion channel function Changes in neuromodulators and neurotransmitters Receptor desensitization | Drugs that work on GABA transmission Drugs that work on Na+, K+, Ca++ ion channels |
Seconds–minutes | Receptor trafficking (relocation from and into the synapse) Alterations in excitability secondary to changes in excitatory and inhibitory receptors at synapse BBB dysfunction | Drugs that work on GABA transmission Drugs that work on carbonic anhydrase and synaptic vesicle protein 2A |
Minutes–hours | Changes in neuropeptide modulators of excitability Often maladaptive changes with overall increase in excitatory (substance P) and decrease in inhibitory (substance Y) peptides causing overall increase in excitability | Downstream effects are overall excitation |
Hours–days/weeks | Long-term effects in gene expression (i.e., inflammatory genes) BBB dysfunction persists Inflammation | Gene modification therapy Anti-inflammatory medications |
Studies of the Transition from Single Seizures to SE: NMDAR
The self-perpetuating nature of SE suggests that synaptic potentiation may account for some of the maintenance mechanisms of SE. Indeed, we found that SE is accompanied by increased long-term potentiation (LTP) in the perforant path-dentate gyrus pathway [45]. Several mechanisms may underlie facilitation of LTP during SE. SE-induced loss of GABA inhibition, which occurs at a very early stage of stimulation, may contribute to facilitation of LTP. However, direct changes affecting excitatory NMDA receptors seem to also be involved. We compared 4–8-week-old rats in self-sustaining SE for 1 h to controls [46]. Physiological measurements included NMDA miniature excitatory postsynaptic currents (mEPSCs) recorded from granule cells in the hippocampal slice with visualized whole-cell patch (Fig. 1.2e). The mEPSCs showed an increase in peak amplitude from −16.2 ± 0.4 pA for controls to −19.5 ± 2.4 for SE (p < 0.001). No significant changes in event decay time were noted. A slight increase in mEPSC frequency was noted for SE cells (1.15 ± 0.51 Hz vs. 0.73 ± 0.37 Hz; 0.05<p < 0.01). Mean–variance analysis of the mEPSCs showed an increase from 5.2 ± 1.2 receptors per synapse in controls to 7.8 ± 2 receptors during SE (50% increase; p < 0.001). Immunocytochemical analysis with antibodies to the NR1 subunit of NMDA receptors showed a movement of NR1 subunits from cytoplasmic sites to the neuronal surface and an increase in colocalization with the presynaptic marker synaptophysin, suggesting a mobilization of “spare” subunits to the synapse (Fig. 1.2f, g).
In conclusion, during SE, endocytosis/internalization of GABAA postsynaptic receptors is accompanied by an increase in excitatory NMDA synaptic receptors. Receptor trafficking may regulate the balance between excitatory and inhibitory postsynaptic receptor numbers and may be an important element in the transition to and maintenance of SE (Fig. 1.3, Table 1.1).
Chemical Models of SE: Kainic Acid
Kainic acid is a naturally occurring algal neurotoxin that activates excitatory kainate-type glutamate receptors and causes seizures in marine mammals and birds up the food chain. This model has been used since the observation was made that the injection of kainate generates repetitive seizures and causes damage in hippocampal neurons [13]. This model and others lead to generation of chronic seizures following the initial SE. SE is induced by giving kainate either systemically (i.e., intravenously, intraperitoneally) or intracranially (i.e., intraventricularly, intrahippocampally). Kainic acid can be administered as a large single dose or smaller doses given repetitively [13]. Most experiments are performed on standard laboratory rats, but other animals have been used, including both male and female mice and dogs. Recordings from the hippocampal and cortical leads will show spikes and repetitive clinical and subclinical (electrographic) seizures and SE. These animals usually go on to develop chronic epilepsy with spontaneous convulsive and non-convulsive seizures. Pathological changes seen following kainate administration resemble those seen in human patients with temporal lobe epilepsy (TLE) and mesial temporal sclerosis (MTS) and include neuronal cell loss and gliosis. As with prior models, these animals can be used to test potential AED treatment in SE, as well as effects on behavior and on induction and course of chronic epilepsy.
Chemical Models of SE: Nerve Agents
Soman, or GD, is an organophosphate (pinacolyl methylphosphonofluoridate) that inactivates acetylcholinesterase, thus causing increased acetylcholine concentration in the central and peripheral nervous system synapses; this leads to induction of SE as well as to salivary hypersecretion, neuromuscular junction block, depressed respiration, and death. SE induces neuroinflammation leading to neuronal cell death and gliosis in the piriform cortex, hippocampus, amygdala, and thalamus [47]. Rat models have been mostly used, and in most animals, epileptiform activity continues for 4 h and in some survivors lasts up to 24 h.
Similar to the pilocarpine model of SE, the soman model can be used to study the role of the cholinergic system in SE. Unlike the pilocarpine model, however, organophosphate administration leads to alteration in nicotinic receptor signaling in addition to the muscarinic receptors. Moreover, GABAergic and glutamatergic systems have been shown to play an important role in this model, once again supporting the complex physiology of SE [48, 49].
Sarin, or GB, is an organophosphate developed in Germany in 1938. It is a clear and odorless liquid which constitutes a weapon of mass destruction, according to the Centers of Disease Control and Prevention [50]. When administered at high doses, sarin causes seizures and respiratory suppression in humans [51]. It was used by terrorists in Japan in 1994 and 1995 in a subway attack. The epidemiological consequences of the 1994 exposure were analyzed and revealed hundreds of affected people including seven deaths [52].
In Vitro Models Used to Study Basic Physiology of SE
Here we will describe a few commonly used techniques used to study molecular, cellular, and network changes, resulting from SE; a more detailed review of basic science techniques is beyond the scope of this chapter.
Brain Slices
A special preparation of brain tissue, termed brain slices [57], is used to study basic physiology of SE and neural tissue in general. Slice preparation allows investigators to answer questions, which would otherwise be difficult to address in vivo. This preparation gives access to deep brain structures and allows one to study tissue properties in the context of preserved local networks. Depending on a study question, a researcher can slice a whole brain or a structure of interest, i.e., hippocampus.
Slice preparations are used to look at acute or chronic changes: acute, by inducing epileptiform discharges directly in the slice, and chronic by using brains from animal models of SE described in prior sections. Slices, once prepared, are then manipulated using electrical or pharmacological methods . Composition of the perfusion solution is usually altered in order to address specific questions.
The following models are used to study physiology of SE [58]:
4-Aminopyridine Model
4-Aminopyridine (4-AP) is a potassium channel blocker that mainly acts on presynaptic sites to decrease repolarization of cell membranes. Administration of 4-AP reproducibly induced epileptiform discharges in in vitro preparations and has been reported to be a proconvulsant in humans. Its effect at the network circuitry has been attributed to enhancement of the glutaminergic tone and neutralization of the GABAergic inhibition [59].
In hippocampal slices, stimulating electrode is usually positioned in the dentate gyrus, and extracellular recordings are made via a recording electrode in CA3, while the slice is continuously perfused with artificial cerebrospinal fluid (aCSF) containing 4-AP. Bipolar stimulating electrode is used to induce spontaneous epileptiform bursting, which starts within 5 min following application of 4-AP and disappear following its removal. In a recent paper, Salami et al. studied effects of 4-AP on high-frequency oscillations (HFOs) showing correlation between presence of HFOs and seizure progression to SE [60].
Low Magnesium Model
This model is used in entorhinal–hippocampal slices while testing effects of drugs on epileptic discharges by measuring extracellular field potential recordings in areas of interest, i.e., the entorhinal cortex or CA1. In these slices, epileptiform activity evolves over time and becomes resistant to BDZ. Similarly to 4-AP model, effects of potential antiepileptic on extracellular field recordings and single-cell physiology can be studied in real time; Heinemann et al. used this model to study effects of SE on energy metabolism and cell survival and showed that calcium played an important role in coupling mitochondrial ATP production to ionic homeostasis [61].
High Potassium Model
Solution containing high potassium evokes epileptiform discharges [62]. Different concentrations of potassium are needed to evoke this activity in different areas, and its effects are studied by using extracellular field recording and whole-cell techniques. Furthermore, lowering extracellular calcium concentration or blocking synaptic transmission in addition to high potassium leads to induction of long-lasting ictal patterns [63, 64].
Organotypic Slice Culture Model
Obtained from neonatal rodents, this preparation allows for slices to be kept in culture for weeks, while cells continue to differentiate eventually producing tissue organization similar to that in situ [65, 66]. However, the circuitry is modified by mossy fiber sprouting and other factors. Just as other slice models, this one can be used to study physiology of SE using EP and intracellular techniques described above. This model is unique in that it allows slices to be kept for longer periods of time thus allowing one to study long-term changes in physiology in the absence of acute trauma and chronic drug effects including effects of reactive oxygen species [67].
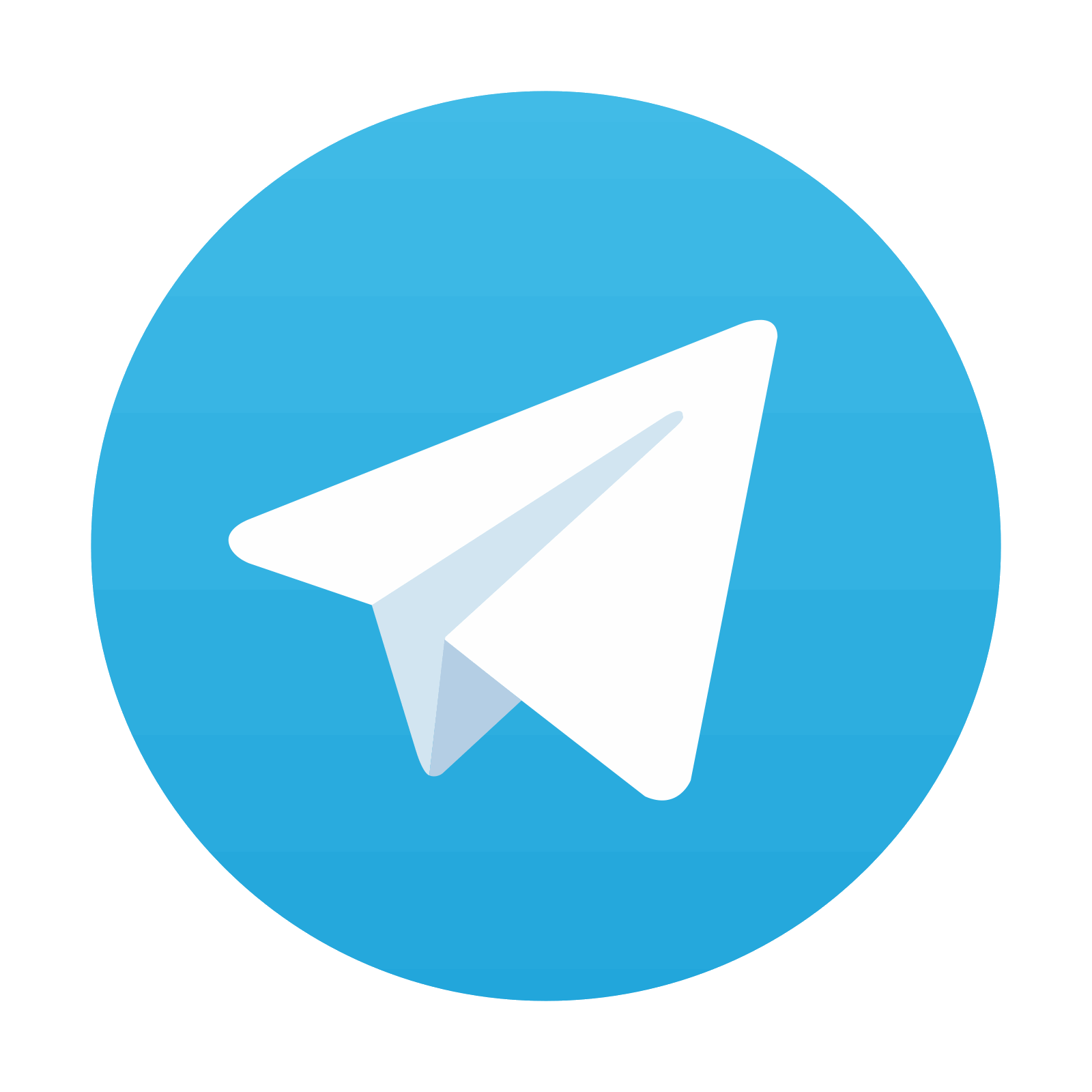
Stay updated, free articles. Join our Telegram channel
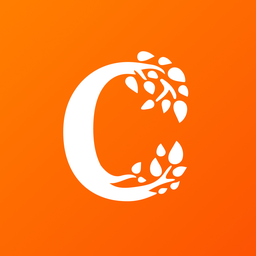
Full access? Get Clinical Tree
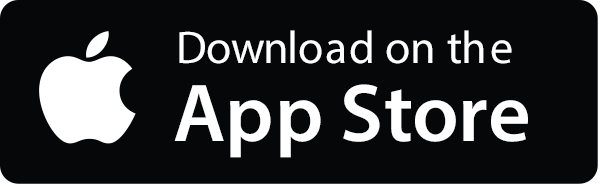
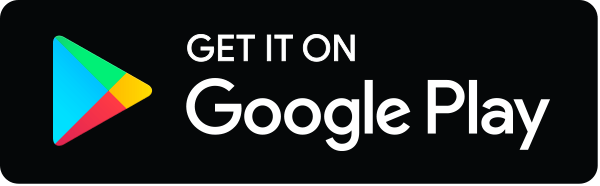