Fig. 1
Three distinct stages of memory as illustrated by two commonly used learning paradigms. Panel a shows the three different stages of memory for contextual fear conditioning . Rodents are allowed to explore a training context and are subjected to a mild electrical shock. During this acquisition stage, a memory for context–shock association is encoded. After training, the animals are returned to the home cage and the consolidation stage starts in which the newly formed memory is consolidated into a long-lasting memory. The memory for the context–shock association is retrieved by re-exposing the animals to the training context, and memory is assessed by measuring freezing behavior (the lack of locomotor activity except for respiratory behavior). Fear conditioning often results in a robust memory for the context–shock association after a single shock presentation, which allows these stages of memory to be isolated in time. b In case of object-location memories , the first stage entails the exploration of a context with two or three distinct objects in specific locations. At the end of the training, the animals are returned to the home cage and the new memory for the object locations is consolidated into a stable long-term memory. The memory for the object locations is tested by re-exposing the animals to the training context but now with one object moved to a novel location. If the animal formed a proper memory for the original location of each object, it will notice that one object was moved and will spend more time exploring the relocated object relative to the non-relocated objects as rodents have the natural tendency to explore familiar object in novel locations
4 Sleep and Fear Memories
Fear conditioning , recently also referred to as threat detection, is a popular learning paradigm to study the encoding and consolidation of fear memories in rodents and serves as a model to elucidate the molecular underpinnings of negative emotional memories (Fig. 1a). Rodents are trained to associate a neutral stimulus such as a context or tone (referred to as the conditioned stimulus, CS) with an aversive stimulus, which most commonly is a mild electrical shock (referred to as the unconditioned stimulus, US). During contextual fear conditioning , the experimental room and test box in which the shock is delivered serve as the US, and this form of fear conditioning requires an intact hippocampus (LeDoux 2000; Maren 2001). Tone-cued fear conditioning is a modified version of the same task in which a tone serves as the CS that is played prior to and during the delivery of the mild electrical shock. The formation of a tone–shock association critically relies on the amygdala rather than the hippocampus (LeDoux 2000; Maren 2001). Memory is most often tested about 1 or 24 h after the conditioning procedure, depending on whether one wants to assess short-term or long-term fear memory formation. Behavioral freezing levels are measured, that is, the complete lack of locomotor activity with the exception of respiratory movement, and higher levels of freezing are used as an indication of a stronger CS-US association. The elegance of this one-trail learning paradigm is that it allows researchers to dissect the role of sleep in the encoding and consolidation of fear memories by manipulating sleep prior to training or directly after training, respectively, as sleep deprivation post-training will only affect memory consolidation.
Several studies in laboratory rats have shown that extended (REM) sleep deprivation for 1–4 days prior to acquisition impairs subsequent encoding and/or formation of contextual fear memories (McDermott et al. 2003; Ruskin et al. 2004; Chen et al. 2006; Tiba et al. 2008; Fernandes-Santos et al. 2012). In one study, prolonged sleep deprivation had no effect on the acquisition of tone-cued fear conditioning (Ruskin et al. 2006). An important implication of this latter finding is that, apparently, sleep deprivation does not have a generalized effect on memory processes but impacts more strongly on specific pathways and brain regions. It seems that encoding of fear memories is more easily impaired by sleep deprivation when it involves the hippocampus, as is the case with contextual fear conditioning, but not so much when it only depends on the amygdala, as is the case with tone-cued fear conditioning. Another implication of these results is that the sleep deprivation-induced memory impairments are not likely a non-specific consequence of fatigue and decreased alertness since the animals were still able to process and use tone-cued information to encode memories. In fact, even with reduced volume cues (down to approximately 10 dB above background noise), 24 h of sleep deprivation failed to impact the encoding of tone-cued fear memories (Ruskin and LaHoste 2009).
In some of the work described in the previous paragraph, rats were subjected to prolonged sleep deprivation by means of the flowerpot or platform-over-water method. To assess whether the impairments in contextual fear conditioning were a consequence of stress hormones, one study included groups with surgically removed adrenals, the main source of glucocorticoid stress hormones . Adrenalectomy in these animals did not prevent the sleep deprivation-induced memory impairments suggesting that elevated corticosterone release as a result of sleep deprivation was not the main cause of the cognitive deficits associated with loss of sleep (Tiba et al. 2008).
With respect to the role of sleep in the consolidation stage, work by different laboratories has clearly shown that depriving animals of sleep for 5–6 h directly after training in the contextual version of the task impairs the formation of contextual fear memories (Graves et al. 2003; Vecsey et al. 2009; Hagewoud et al. 2010a, 2011). Rats or mice were transferred to the shock box, exposed to a mild foot shock, and then returned to their home cage where they were sleep deprived for 5–6 h. The next day, generally 24 h after the conditioning, animals were re-exposed to the shock box to test their memory. During the re-exposure, the animals that had been sleep deprived displayed a significantly diminished freezing response as compared to the control animals that had not been sleep deprived. This finding suggests that loss of sleep during the first few hours after the training negatively impacted memory consolidation. Importantly, in between the sleep deprivation and the re-exposure to the shock box, all animals had sufficient time to sleep and recover, so the behavioral deficits during the re-exposure test could not be explained by fatigue. Also, the impairment was not observed when the sleep deprivation window was delayed for 5 h (i.e., sleep deprivation from 5 to 10 h after training rather than 0–5 h after training), which confirms that the effects of immediate sleep deprivation upon memory next day was not a consequence of remaining fatigue (Graves et al. 2003). The latter finding further suggests that there is a specific time window after acquisition during which memory formation is supported by sleep and can be disrupted by sleep deprivation (Graves et al. 2003).
In contrast to hippocampus-dependent contextual fear memories, the consolidation of tone-cued fear memories does not require the hippocampus but, rather, depends on the amygdala. This form of conditioning is not disrupted by a brief period of sleep deprivation following training (Graves et al. 2003). In agreement with the findings on sleep deprivation prior to memory encoding, this observation suggests that memory consolidation is particularly susceptible to sleep deprivation or sleep disruption when it involves the hippocampus and less so when it depends mostly on the amygdala (Graves et al. 2003).
The interference theory of forgetting implies that processing newer information interferes with storing and remembering previously learned information (retroactive interference) (Robertson 2012). The cited studies on brief sleep deprivation and contextual fear conditioning kept animals awake by means of the gentle stimulation or gentle handling method. It is a debate in the field of sleep research whether the reported memory deficits are caused by the stimulation the animals receive and the interference that may cause or by the lack of sleep per se. Recent work by Hagewoud and colleagues addressed this topic. The authors first conducted a contextual fear conditioning experiment during the light phase (i.e., subjective night) as described above and recorded the amount of stimulation that was necessary to keep animals awake for the first 6 h directly after training (Hagewoud et al. 2010a). Next, the authors repeated the fear conditioning experiment during the dark phase (i.e., subjective day) and applied the exact same amount of stimulation over 6 h that was required to keep animals awake for 6 h during the light phase regardless of whether the animal were already spontaneously awake or trying to sleep. This amount of stimulation when applied during the dark phase did not impair memory consolidation. This important finding indicates that the sleep deprivation-induced fear memory deficits observed when training and sleep deprivation was conducted in the light phase were not a consequence of the method used to deprive animals of sleep, but a result of the actual loss of sleep. In contrast to 6 h of sleep deprivation during the dark phase, 12 h of sleep deprivation in the dark phase did hamper the long-term memory formation of contextual fear. Because sleep is more spread out across the dark phase, the authors concluded that the amount of sleep in addition to sleep during a specific time window after training is another important factor for memory consolidation (Hagewoud et al. 2010a).
5 Sleep, Object-Place Memories, and Object-Identity Memories
Object memory tasks are based on the rodent’s natural tendency to explore spatial novelty (Fig. 1b). Generally, the animals are transferred to a test room and allowed to freely explore a context equipped with a number of unique objects during an initial training session (acquisition phase). After a certain period of time, which can range from hours to days, the animals are returned to the test box (test phase) in which one object has been moved to a new location in the box or has been replaced by a new one. The animals then have to discriminate familiar from novel objects (object-identity recognition task ) or determine which familiar object has moved to a novel location (object-location memory task ). Their ability to discriminate the familiar objects or locations from novel objects or locations is reflected in their preferential exploration of the novel objects and location. As with fear conditioning, the elegance of these behavioral assays lies in the fact that they are brief, one-session learning tasks allowing for easy manipulation of sleep either prior or after training. In addition, these paradigms have great translational potential because versions of the same task can be used in both animal models and humans.
While to our knowledge, there have been no studies on sleep deprivation prior to the acquisition phase in the object-identity or object-location memory paradigms, various studies have assessed the effects of sleep deprivation immediately following training. The object-location memory task critically depends on the hippocampus (Winters et al. 2004; Oliveira et al. 2010), and 5–6 h of sleep deprivation directly after training impairs the memory consolidation for object location. When mice are re-tested in the task 24 h after conditioning, they fail to discriminate the relocated from non-relocated objects (Florian et al. 2011; Havekes et al. 2014). A recent study further refined the window during which sleep deprivation impacted memory consolidation in this task by showing that 3 h of sleep deprivation significantly impaired memory formation when deprivation began 1 h after training. In contrast, 3 h of deprivation beginning immediately post-training did not impair spatial memory (Prince et al. 2014; also, see Fig. 2).
In addition to object-location memories, a number of studies have indicated that 5–6 h of brief sleep deprivation directly after acquisition using the mild stimulation method significantly reduced the preferential exploration of a novel object relative to familiar objects the next day. These findings indicate that the consolidation of object-identity memories just like the consolidation of object-location memories is negatively impacted by sleep deprivation (Palchykova et al. 2006; Halassa et al. 2009). Using an optogenetic approach to wake up mice by activating orexin/hypocretin neurons, the laboratory of de Lecea recently found that the consolidation of memories for object identity is attenuated by sleep fragmentation during the first 4 h after training (Rolls et al. 2011). When the average duration of sleep episodes was maintained at around 70 % of normal, the memory for the object identity was unaffected. An important implication of this study is that in addition to total sleep deprivation even subtler manipulations such as mild sleep fragmentation may already lead to memory impairments. Secondly, because the optogenetic method of sleep disruption does not require handling or physical stimulation of the animals, it strongly supports the view that the memory deficits are a consequence of an impairment or loss of sleep per se rather than the stimulation that is provided to keep the animals awake.
There is some controversy regarding the involvement of the hippocampus in the formation of memories for object identities. Some studies indicate that the perirhinal cortex rather than the hippocampus is critical for the consolidation of object identities (Brown and Aggleton 2001; Winters and Bussey 2005; Oliveira et al. 2010), while others suggest that the hippocampus is critical for this process (Cohen et al. 2013). Recent work addressed this issue and revealed that when the number of objects in the task was increased (thus making the task more difficult) it became more and more dependent on the hippocampus (Sannino et al. 2012). Because sleep deprivation also impairs memory consolidation of object identity using a simple version of the task that does not require the hippocampus, other regions such as the perirhinal cortex may also be susceptible to sleep loss (Halassa et al. 2009; Oliveira et al. 2010).
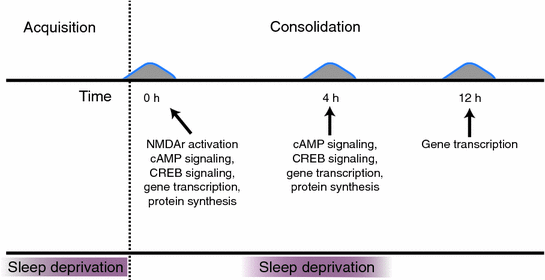
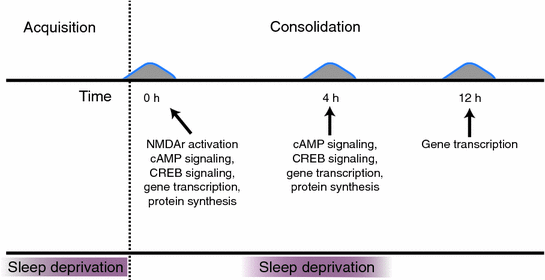
Fig. 2
Sleep deprivation can affect different phases of memory. Sleep deprivation prior to learning can impair memory acquisition, and sleep deprivation after training can have a negative effect on memory consolidation. During the consolidation phase, there are multiple time windows in which specific molecular processes contribute to the formation of a stable long-term memory. During the first window, directly after training at the beginning of memory consolidation, these include NMDA receptor activation, cAMP signaling, CREB-mediated gene transcription, and the synthesis of novel proteins. During the second period, approximately 4 h after training, there is a second wave of cAMP signaling, CREB-mediated gene transcription, and protein synthesis. Gene transcription 12 h after training also contributes to the memory consolidation process. In case of the object-location memory task, sleep deprivation specifically impacts memory consolidation during a 3 h window starting 1 h after training (i.e., from 1 to 4 h after training)
6 Sleep, Spatial Memories, and Behavioral Strategies
The formation of spatial memories , just like contextual fear and object-location memories, requires proper hippocampal function. To study spatial learning in rodents, a variety of behavioral paradigms have been developed. One of the most frequently used assays to study spatial and non-spatial learning and memory processes in rodents is the Morris water maze (Morris et al. 1982). The essence of this task is that animals are placed in a large pool of opaque water and learn that they can escape from the water by finding a submerged platform. In the hippocampus-dependent spatial version, the animals have to learn to locate the platform on the basis of spatial cues that are present around the pool. The task is made hippocampus-independent by a single visual cue such as a flag directly marking the specific location of the platform. In contrast to the fear conditioning and object tasks, the water maze is a more complex, multi-trial learning task and often animals are subjected to multiple training sessions on successive days.
Animals that were deprived of REM sleep using the flowerpot method for 12 h directly after training had greater difficulty learning platform location reflected in a longer latency time to find the platform in the water maze. Delaying the REM sleep deprivation period for 12 h did not affect memory consolidation suggesting that the memory deficit in the first experiment was due to hampered memory consolidation rather than fatigue as a result of sleep loss (Smith and Rose 1996). In line with this, even as little as 4 h of REM sleep deprivation immediately following training was sufficient to impair this form of memory (Smith and Rose 1996).
The observation that REM sleep is critical for the spatial learning in the Morris water maze has been confirmed by some laboratories (Youngblood et al. 1997; Guan et al. 2004; Yang et al. 2008; Li et al. 2009), but not by some others (Wang et al. 2009; Walsh et al. 2011). The apparent inconsistent findings on the consequences of sleep deprivation in the water maze may be explained by the fact that animals while undergoing training can adopt alternative behavioral learning strategies that are not necessarily dependent on the hippocampus (Bjorness et al. 2005; Hairston et al. 2005). Instead of locating the platform on the basis of the spatial cues in the testing room, animals may confer to using other strategies that do not rely on those cues. In other words, even when sleep deprivation presumably impaired hippocampal function, the animals were still able to learn the location of the platform using more random behavioral strategies based on different brain mechanisms. An important implication of these findings is that effects of sleep deprivation in the brain, even when they are there, may not always be directly evident at the behavioral or cognitive level.
The observation that loss of sleep can lead to the use of alternative behavioral strategies has also been reported by others using different spatial learning paradigms, including the 8-arm radial maze task (Bjorness et al. 2005) and the y-maze or T-maze task (Hagewoud et al. 2010b). Using a two-arm reference task in which animals have to learn and remember the location of a food reward, Hagewoud and colleagues (2010b) showed that sleep deprivation directly following each training session did not directly affect the rate at which mice learned which arm was baited. However, sleep deprivation did induce a shift in the behavioral strategy used to locate the food reward. Whereas a majority of control animals used a spatial strategy based on cues in the room to locate the baited arm, the sleep-deprived mice learned to make a specific turning response, independent of spatial cues. The latter was demonstrated at the end of the training phase, when all animals had successfully learned to locate the baited arm, by basically turning the T-maze 180° and subjecting the animals to a probe trial starting from the opposite spatial location. Despite the altered spatial location of the start arm, the majority of control animals went to the original and correct spatial location for the food reward. Instead, the sleep-deprived animals made the same turn as they had learned to do and therefore went into the opposite arm. Thus, instead of a spatial strategy , the sleep-deprived mice used a so-called response-based strategy , which is known to be dependent on the striatum rather than the hippocampus (Hagewoud et al. 2010b). By doing so, the mice presumably compensated for a sleep deprivation-induced hippocampal deficit by recruiting brain regions apparently less sensitive to sleep loss. Indeed, analysis of brain material showed that the shift in behavioral strategy was paralleled by a shift in regional brain activation from hippocampus to striatum (Hagewoud et al. 2010b). The observation that sleep deprivation may facilitate of striatum-dependent learning has also been suggested by other studies (Watts et al. 2012).
Importantly, while sleep-deprived mice in these maze studies by Hagewoud and colleagues performed well during the initial training, their performance was attenuated when they had to adapt their memory in a subsequent reversal-learning paradigm. In this reversal-learning task, the mice had to learn that the previously rewarded arm was no longer rewarded and that the previously non-rewarded arm was now rewarded. The sleep-deprived mice required significantly more training sessions to adapt to this changed situation than the control mice (Hagewoud et al. 2010b). The authors explained this outcome by the well-known rigidity of the striatal memory system as compared to the more flexible hippocampal memory system used by the control animals (Hartley et al. 2003).
Together, these findings have two important implications. One, when the nature of the task allows this, the negative effects of sleep deprivation on hippocampal function and cognition may be temporarily compensated for through the recruitment of other brain regions and alternative learning strategies . Two, the use of such alternative learning mechanisms can result in reduced flexibility, which may still have negative consequences for performance later on, when changing conditions require adaptation of the previously formed memories. These observations in rodents parallel imaging work in humans showing shifts in activity of specific brain regions as a consequence of sleep deprivation (Orban et al. 2006; Rauchs et al. 2008).
7 Sleep Deprivation and Working Memory
Certain spatial learning paradigms have also been used to assess more specifically the effects of sleep deprivation on working memory . One task that is particularly well suited to address this question is the novel-arm recognition task in which rodents have to discriminate a novel arm from familiar ones that have been explored two minutes prior to the test, a form of working memory that also critically depends on the hippocampus , but not the prefrontal cortex (Etkin et al. 2006). Non-sleep-deprived mice preferentially explored the novel arm during the test session suggesting that they formed a short-term memory for those arms they already explored during training. In contrast, mice sleep deprived for 12 h prior to exposure to the task did not discriminate between familiar and novel arms and explored all arms to a similar level due to the lack of an accurate short-term memory for the previously explored arms. Mice that were deprived of sleep for 6 h showed an intermediate phenotype (Hagewoud et al. 2010c). The deficit in working memory was not a consequence of reduced motivation due to fatigue because the total number of arm entries was not affected by sleep loss. These findings are conform studies in humans that have indicated that a single night of sleep deprivation or even mild sleep disruption is sufficient to impair the memory encoding process that coincides with reduced hippocampal activity (Yoo et al. 2007; Van Der Werf et al. 2009).
8 Sleep Deprivation and the Molecular Machinery Underlying Memory Consolidation
The emerging picture from the behavioral studies in animals described in the previous paragraphs is that those cognitive processes that require the hippocampus benefit from sleep and are particularly sensitive to sleep loss. The true value of these animal models of course lies in the fact that they can be used for experimentation and detailed analysis of underlying cellular and molecular mechanisms that cannot be done in human subjects. In the next section, we will describe recent insights in the molecular signaling pathways critical for the consolidation of hippocampus-dependent long-term memories and discuss how sleep and sleep loss alters these processes (for an overview, see Fig. 3).
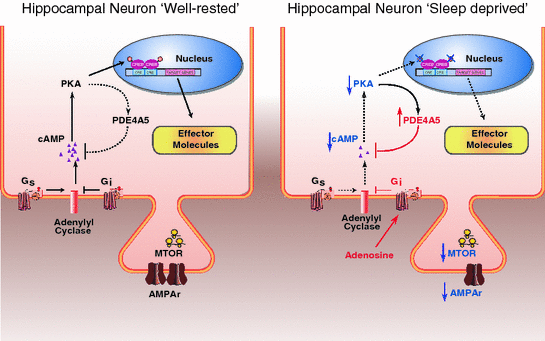
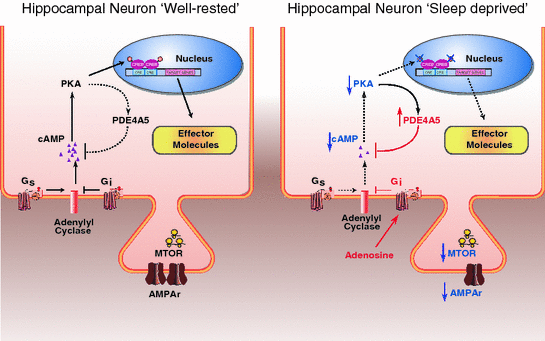
Fig. 3
The molecular impact of sleep deprivation on pathways critical for memory consolidation . A schematic overview of some of the hippocampal signaling pathways whose modulation by sleep deprivation may contribute to the effects of sleep deprivation on memory encoding and consolidation. Left Panel signaling pathways under normal well-rested conditions. Right Panel sleep deprivation has been reported to reduce cAMP signaling leading to reduced AMPA receptor phosphorylation and CREB -mediated gene transcription. Sleep loss also hampers translation initiation through the mTOR pathway. Dashed black lines and blue arrows pointing down indicate attenuation of the signaling pathway. Red labels and lines indicate an increase of the signaling pathway in the sleep deprived condition relative to the well-rested condition
8.1 Sleep Deprivation Attenuates cAMP Signaling
Time course analysis of the memory consolidation period revealed that not one, but two critical time periods exist during which hippocampal mRNA synthesis and protein synthesis critically contributes to fear motivated learning (Bourtchouladze et al. 1998; Igaz et al. 2002). One of the pathways that modulate transcriptional processes during these two periods is the cAMP signaling pathway with PKA being one of the major targets of cAMP. Indeed, the formation of long-term spatial and contextual fear memories is disrupted by forebrain-specific suppression of PKA function (Abel et al. 1997; Isiegas et al. 2006) and by infusion of the PKA inhibitor Rp-cAMP bilaterally into the hippocampus (Bourtchouladze et al. 1998). Importantly, Rp-cAMP disrupts long-term fear memory formation when it is injected either directly after training or 4 h post-training. Because injections of the PKA inhibitor at other time points after training did not lead to memory deficits, the authors concluded that PKA signaling in the hippocampus contributes to the memory consolidation process in the first few hours after training (Bourtchouladze et al. 1998). This finding is of particular interest in light of the studies discussed in previous sections showing that hippocampus-dependent memory formation is impaired by sleep deprivation during a similar time window (Graves et al. 2003; Hagewoud et al. 2010a, 2011; Florian et al. 2011; Havekes et al. 2014). In case of object-location memories , this time window has even been refined to 2–4 h after training (Prince et al. 2014). The similarity in time windows during which sleep deprivation and PKA-inhibition disturb memory consolidation raised the question whether hippocampal cAMP-PKA signaling is raised during sleep and suppressed by sleep deprivation. Several reports have provided evidence that this may indeed be the case. Recent work revealed that hippocampal cAMP signaling undergoes circadian oscillations with a peak around 4 h after the onset of the light period (Eckel-Mahan et al. 2008), which corresponds to the period in the light phase during which mice spent a significant time sleeping (Wimmer et al. 2013). Furthermore, hippocampal cAMP signaling is increased specifically during rapid eye movement sleep in the mouse hippocampus (Luo et al. 2013). Work by our laboratory revealed that loss of sleep has the inverse effect on the cAMP signaling pathway in the hippocampus; five hours of total sleep deprivation reduces cAMP levels in the hippocampus and impaired cAMP-dependent forms of synaptic plasticity that require PKA signaling (e.g., LTP induced by four spaced 100 Hz trains, thetaburst stimulation, or bath application with the adenylate cyclase activator forskolin) (Vecsey et al. 2009).
Subsequent studies showed that transiently increasing cAMP levels in hippocampal excitatory neurons during the course of sleep deprivation can prevent the memory deficits in some of the learning paradigms. Havekes and colleagues (2014) used a pharmacogenetic approach based on the expression of Drosophila octopamine receptors , which are normally not present in the mammalian brain, specifically in the hippocampus of mice. Brain region-specific expression of the octopamine receptor was achieved by injecting a virus with the gene construct directly into the hippocampus. Expression of the receptor was restricted to excitatory neurons by using a CaMKIIα promoter fragment to drive transgene expression. Once the receptors were expressed, a simple intraperitoneal injection of the receptor ligand octopamine binding to the receptors was capable of activating adenylyl cyclases leading to a transient increase in cAMP . The authors then trained animals expressing the octopamine receptor or control animals expressing eGFP in the object-place recognition task and sleep deprived half of these groups for 5 h directly after training. All mice received two systemic injections with octopamine to boost cAMP production and prevent the previously reported decrease in cAMP levels during sleep deprivation. This transient upregulation of cAMP signaling selectively in hippocampal excitatory neurons was sufficient to prevent the memory impairments in the object-location memory task seen in sleep-deprived control animals. These studies thus provided evidence that local changes in cAMP in hippocampal neurons as a result of sleep deprivation during the consolidation window lead to memory deficits in a hippocampus-dependent task (Havekes et al. 2014).
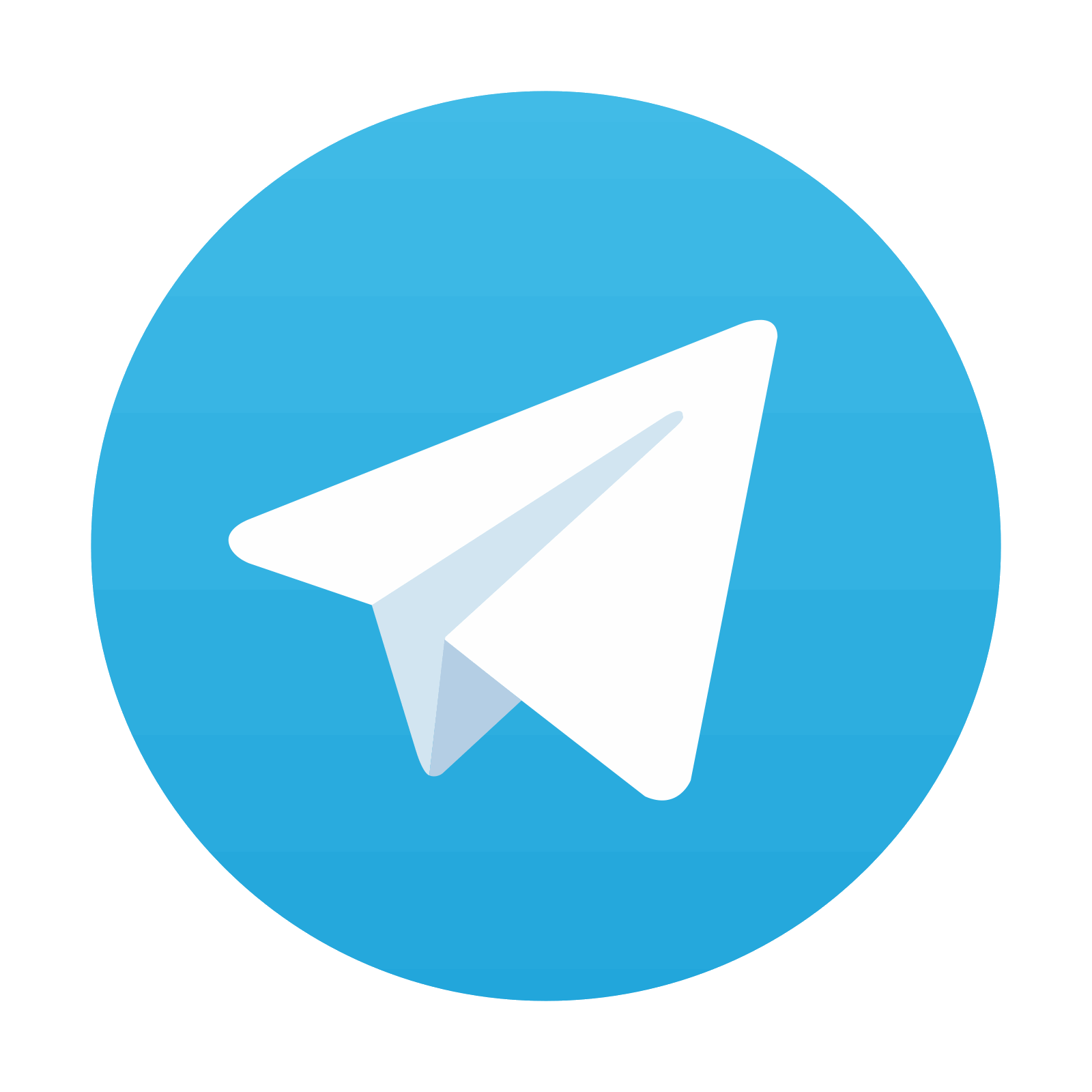
Stay updated, free articles. Join our Telegram channel
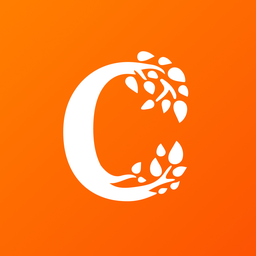
Full access? Get Clinical Tree
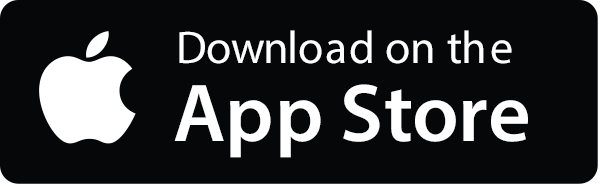
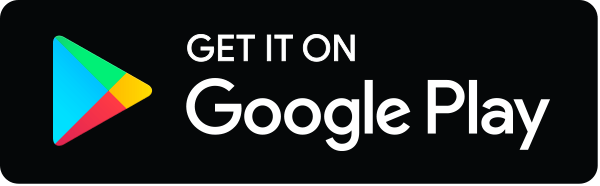