Synaptic Integration in the Central Nervous System
Central Neurons Receive Excitatory and Inhibitory Inputs
Excitatory and Inhibitory Synapses Have Distinctive Ultrastructures
The Excitatory Ionotropic Glutamate Receptors Are Encoded by a Distinct Gene Family
NMDA and AMPA Receptors Are Organized by a Network of Proteins at the Postsynaptic Density
Currents Through Single GABA and Glycine Receptor-Channels Can Be Recorded
Ionotropic GABAA and Glycine Receptors Are Homologous to Nicotinic ACh Receptors
Some Synaptic Actions Depend on Other Types of Ionotropic Receptors in the Central Nervous System
Excitatory and Inhibitory Synaptic Actions Are Integrated by the Cell into a Single Output
Synaptic Inputs Are Integrated to Fire an Action Potential at the Axon Initial Segment
Dendrites Are Electrically Excitable Structures That Can Fire Action Potentials
Synapses on a Central Neuron Are Grouped According to Physiological Function
LIKE SYNAPTIC TRANSMISSION at the neuromuscular junction, most rapid signaling between neurons in the central nervous system involves ionotropic receptors in the postsynaptic membrane. Thus, many principles that apply to the synaptic connection between the motor neuron and skeletal muscle fiber at the neuromuscular junction also apply in the central nervous system. Synaptic transmission between central neurons is more complex, however, for several reasons. First, although most muscle fibers are innervated by only one motor neuron, a central nerve cell (such as the motor neuron in the spinal cord) receives connections from hundreds or even thousands of neurons. Second, muscle fibers receive only excitatory inputs, whereas central neurons receive both excitatory and inhibitory inputs. Third, all synaptic actions on muscle fibers are mediated by one neurotransmitter, acetylcholine (ACh), which activates only one type of receptor (the ionotropic nicotinic ACh receptor); however, a single central neuron can respond to different types of inputs, each mediated by a distinct transmitter that alters the activity of specific types of receptor. These receptors include both ionotropic receptors, where binding of transmitter directly opens an ion channel, and metabotropic receptors, where transmitter binding indirectly regulates a channel by activating second messengers. As a result, unlike muscle fibers, central neurons must integrate diverse inputs into a single coordinated action. Finally, the nerve–muscle synapse is a model of efficiency—every action potential in the motor neuron produces an action potential in the muscle fiber. In comparison, connections made by a presynaptic neuron onto the motor neuron are only modestly effective—often 50 to 100 excitatory neurons must fire together to produce a synaptic potential large enough to trigger an action potential in a motor cell.
The first insights into synaptic transmission in the central nervous system came from experiments by John Eccles and his colleagues in the 1950s on the synaptic inputs onto spinal motor neurons that control the stretch reflex, the simple behavior we considered in Chapter 2. The spinal motor neurons remain particularly useful for examining central synaptic mechanisms because they have large, accessible cell bodies and, most important, they receive both excitatory and inhibitory connections and therefore allow us to study the integrative action of the nervous system on the cellular level.
Central Neurons Receive Excitatory and Inhibitory Inputs
To analyze the synapses that mediate the stretch reflex, Eccles activated a large population of axons of the sensory cells that innervate the stretch receptor organs in the quadriceps (extensor) muscle (Figure 10–1A,B). Nowadays the same experiments can be done by stimulating a single sensory neuron. For example, passing sufficient current through a microelectrode into the cell body of a stretch-receptor neuron that innervates the extensor muscle generates an action potential in the sensory cell. This in turn produces a small excitatory postsynaptic potential (EPSP) in the motor neuron that innervates precisely the same muscle (in this case the quadriceps) monitored by the sensory neuron (Figure 10–1B upper panel). The EPSP produced by the one sensory cell, the unitary EPSP, depolarizes the extensor motor neuron by less than 1 mV, often only 0.2 to 0.4 mV, far below the threshold for generating an action potential (typically, a depolarization of 10 mV or more is required to reach threshold).
Figure 10-1 The combination of excitatory and inhibitory synaptic connections mediating the stretch reflex of the quadriceps muscle is typical of circuits in the central nervous system.
A. The stretch-receptor sensory neuron at the extensor (quadriceps) muscle makes an excitatory connection with an extensor motor neuron that innervates this same muscle group. It also makes an excitatory connection with an interneuron, which in turn makes an inhibitory connection with a flexor motor neuron that innervates the antagonist biceps femoris muscle group. Conversely, an afferent fiber from the biceps (not shown) excites an interneuron that makes an inhibitory synapse on the extensor motor neuron.
B. This idealized experimental setup shows the approaches to studying the inhibition and excitation of motor neurons in the pathway illustrated in part A. Above: Two alternatives for eliciting excitatory postsynaptic potentials (EPSPs) in the extensor motor neuron. A single presynaptic axon can be stimulated by inserting a current-passing electrode into the sensory neuron cell body. An action potential in the sensory neuron stimulated in this way triggers a small EPSP in the extensor motor neuron (black trace). Alternatively, the whole afferent nerve from the quadriceps can be stimulated electrically with extracellular electrodes. The excitation of many afferent neurons through the extracellular electrode generates a synaptic potential (dashed trace) large enough to initiate an action potential (red trace). Below: The setup for eliciting and measuring inhibitory potentials in the flexor motor neuron. Intracellular stimulation of a single inhibitory interneuron receiving input from the quadriceps pathway produces a small inhibitory (hyperpolarizing) postsynaptic potential (IPSP) in the flexor motor neuron (black trace). Extracellular stimulation recruits numerous inhibitory neurons and generates a larger postsynaptic IPSP (red trace). (Action potentials from the sensory neuron and interneuron appear smaller because they were recorded at lower amplification than the motor neuron action potentials).
The generation of an action potential requires the near-synchronous firing of a number of sensory neurons. This can be observed in an experiment in which a population of sensory neurons is stimulated by passing current through an extracellular electrode. As the strength of the extracellular stimulus is increased, more sensory afferent fibers are excited, and the depolarization produced by the EPSP becomes larger. The depolarization eventually becomes large enough to bring the membrane potential of the axon initial segment (the integrative component of the motor neuron) to the threshold for an action potential.
In contrast to the EPSP produced in the extensor motor neuron, stimulation of the extensor stretch-receptor neuron produces a small inhibitory postsynaptic potential (IPSP) in the motor neuron that innervates the flexor muscle, which is antagonistic to the extensor muscle (Figure 10–1B lower panels). This hyperpolarizing action is mediated by an inhibitory interneuron, which receives excitatory input from the sensory neurons of the extensor muscle and in turn makes synapses with the motor neurons that innervate the flexor muscle. In the laboratory a single interneuron can be stimulated intracellularly to directly elicit a small unitary IPSP in the motor neuron. Extracellular activation of an entire population of interneurons elicits a larger IPSP.
Although a single EPSP in the extensor motor neuron is not nearly large enough to elicit an action potential, the neuron integrates many EPSPs from a large number of afferent sensory fibers to initiate an action potential. At the same time, IPSPs, if strong enough, can counteract the sum of the excitatory actions and prevent the membrane potential from reaching threshold. In addition to counteracting synaptic excitation, synaptic inhibition can exert powerful control over action potential firing in neurons that are spontaneously active because of the presence of intrinsic pacemaker channels. This function, called the sculpting role of inhibition, shapes the pattern of firing in such cells (Figure 10–2).
Figure 10-2 Inhibition can shape the firing pattern of a spontaneously active neuron. Without inhibitory input the neuron fires continuously at a fixed interval. With inhibitory input (arrows) some action potentials are inhibited, resulting in a distinctive pattern of impulses.
Excitatory and Inhibitory Synapses Have Distinctive Ultrastructures
As we learned in Chapter 8, the effect of a synaptic potential—whether it is excitatory or inhibitory—is determined not by the type of transmitter released from the presynaptic neuron but by the type of ion channels in the postsynaptic cell activated by the transmitter. Although some transmitters can produce both excitatory and inhibitory postsynaptic potentials, by acting on distinct classes of ionotropic receptors at different synapses, most transmitters produce a single predominant type of synaptic response; that is, a transmitter is usually inhibitory or excitatory. For example, in the vertebrate brain neurons that release glutamate typically act on receptors that produce excitation; neurons that release γ-aminobutyric acid (GABA) or glycine act on receptors that produce inhibition.
The synaptic terminals of excitatory and inhibitory neurons can be distinguished by their morphology. Two morphological types are common in the brain: Gray type I and type II (named after E. G. Gray, who described them). Most type I (asymmetric) synapses are glutamatergic and therefore excitatory, whereas most type II synapses (symmetric) are GABAergic and therefore inhibitory. Type I synapses have round synaptic vesicles, an electron-dense region at the active zone of the presynaptic membrane, and an even larger dense region in the postsynaptic membrane apposed to the active zone, known as the postsynaptic density (PSD). Type II synapses have oval or flattened synaptic vesicles with less obvious presynaptic membrane specializations and PSD (Figure 10–3). Although type I synapses are mostly excitatory and type II inhibitory, the two morphological types have proved to be only a first approximation to transmitter biochemistry. As we shall learn in Chapter 13, immunocytochemistry affords much more reliable distinctions between transmitter types based on the biochemical nature of the transmitters or the enzymes involved in their synthesis.
Figure 10-3 The two most common morphological types of synapses in the central nervous system are Gray type I and type II. Type I is usually excitatory, exemplified by glutamatergic synapses; type II is usually inhibitory, exemplified by GABAergic synapses. Differences include the shape of vesicles, prominence of presynaptic densities, total area of the active zone, width of the synaptic cleft, and presence of a dense basement membrane. Type I synapses typically contact specialized projections on the dendrites, called spines, and less commonly contact the shafts of dendrites. Type II synapses often contact the cell body and dendritic shaft.
Excitatory Synaptic Transmission Is Mediated by Ionotropic Glutamate Receptor-Channels That Are Permeable to Sodium and Potassium
The excitatory transmitter released from the presynaptic terminals of stretch-receptor neurons is the amino acid L-glutamate, the major excitatory transmitter in the brain and spinal cord. Eccles and his colleagues discovered that the EPSP in spinal motor cells results from the opening of glutamate-gated channels permeable to both Na+, and K+. This ionic mechanism is similar to that produced by ACh at the neuromuscular junction described in Chapter 9. Like the ACh-gated channels, the glutamate-gated channels conduct both Na+, and K+ with nearly equal permeability. As a result, the reversal potential for current flow through these channels is 0 mV (see Figure 9-7).
Glutamate receptors can be divided into two broad categories: the ionotropic receptors, which are ligand-gated channels where glutamate binding directly opens the channel, and metabotropic receptors, which are G protein-coupled receptors that indirectly gate channels through the production of second messengers (Figure 10–4). There are three major subtypes of ionotropic glutamate receptors: AMPA, kainate, and NMDA, named according to the types of synthetic agonists that activate them (α-amino-3-hydroxy-5-methylisoxazole-4-propionic acid, kainate, and N-methyl-D-aspartate, respectively). The NMDA receptor is selectively blocked by the drug APV (2-amino-5-phosphonovaleric acid). The AMPA and kainate receptors are not affected by APV but both are blocked by the drug CNQX (6-cyano-7-nitroquinoxaline-2,3-dione), and thus they are sometimes called the non-NMDA receptors. The metabotropic glutamate receptors can be selectively activated by trans-(1S,3R)-1-amino-1, 3-cyclopentanedicarboxylic acid (ACPD). The action of ionotropic glutamate receptors is always excitatory or depolarizing, as the reversal potential of their ionic current is near zero, whereas the metabotropic receptors can produce either excitation or inhibition, depending on the reversal potential of the ionic currents that they regulate.
Figure 10-4 Different classes of glutamate receptors regulate excitatory synaptic actions in neurons in the spinal cord and brain.
A. Three classes of ionotropic glutamate receptors directly gate ion channels permeable to cations. The AMPA and kainate type of receptors bind the glutamate agonists AMPA or kainate, respectively. These receptors contain a channel that is permeable to Na+, and K+. The NMDA receptor, which binds the glutamate agonist NMDA, contains a channel permeable to Ca2+, K+, and Na+. It has binding sites for glutamate, glycine, Zn2+, phencyclidine (PCP, or angel dust), MK801 (an experimental drug), and Mg2+, each of which regulates the functioning of the channel differently.
B. The metabotropic glutamate receptors indirectly gate ion channels by activating a GTP-binding protein, which in turn interacts with effector molecules that alter metabolic and ion channel activity (see Chapter 11).
The NMDA receptor has several interesting properties. First, this ligand-gated channel is permeable to Ca2+ as well as to Na+, and K+ (Figure 10-4A). Second, opening the channel requires extracellular glycine as a cofactor. Under normal conditions the concentration of extracellular glycine is sufficient to allow the NMDA receptor-channel to be activated efficiently by glutamate. Third, the NMDA receptor is unique among ligand-gated channels thus far characterized because its opening depends on membrane voltage as well as transmitter. The voltage-dependence is caused by a mechanism that is quite different from that employed by the voltage-gated channels that generate the action potential. In the latter, changes in membrane potential are translated into conformational changes in the channel by an intrinsic voltage-sensor. In the NMDA receptors, however, depolarization removes an extrinsic plug from the channel. At the resting membrane potential (-65 mV) extracellular Mg2+ binds tightly to a site in the pore of the channel, blocking ionic current. But when the membrane is depolarized (for example, by the opening of AMPA receptor-channels), Mg2+ is expelled from the channel by electrostatic repulsion, allowing Na+, and Ca2 to enter (Figure 10–5).
Figure 10-5 Opening of single NMDA receptor-channels depends on voltage in addition to glutamate. These recordings are from individual NMDA receptor-channels (from rat hippocampal cells in culture). Downward deflections indicate pulses of inward (negative) current; upward deflections indicate outward (positive) current. (Reproduced, with permission, from J. Jen and C. F. Stevens.)
A. When Mg2+ is present in normal concentration in the extracellular solution (1.2 mM), the channel is largely blocked at the resting potential (-60 mV). At negative membrane potentials only brief, flickery, inward currents are seen upon channel opening because of the Mg2+ block. Substantial depolarization (to +30 mV or +60 mV) relieves the Mg2+ block, permitting longer-lasting pulses of outward current through the channel.
B. When Mg2+ is removed from the extracellular solution, the opening and closing of the channel do not depend on voltage. The channel is open at the resting potential of -60 mV, and the synaptic current reverses near 0 mV, like the total synaptic current (see Figure 10-6B).
The NMDA receptor has the further interesting property that it is inhibited by the hallucinogenic drug phencyclidine (PCP, also known as angel dust) and by MK801, both of which bind to a site in the pore of the channel that is distinct from the Mg2+ binding site (Figure 10-4A). Indeed, blockade of NMDA receptors produces symptoms that resemble the hallucinations associated with schizophrenia, whereas certain antipsychotic drugs enhance current flow through the NMDA receptor-channels. This has led to the hypothesis that schizophrenia may involve a defect in NMDA receptor function.
At most central synapses that use glutamate as the transmitter, the postsynaptic membrane contains both NMDA and AMPA receptors. The contributions of current through NMDA and AMPA receptors to the total excitatory postsynaptic current (EPSC) can be dissected using pharmacological antagonists in a voltage-clamp experiment (Figure 10–6). At the normal resting potential of most neurons, the NMDA receptor-channels are largely inhibited by Mg2+. As a result, the EPSC is predominantly determined by charge flow through the AMPA receptors, which generate a current with a very rapid rising phase and very rapid decay phase. However, as a neuron becomes depolarized, Mg2+ is driven out of the mouth of the NMDA receptors and more charge flows through these channels. Thus, the NMDA receptor conducts current maximally when two conditions are met: Glutamate is present, and the cell is depolarized (Figure 10–6). That is, the NMDA receptor acts as a “coincidence detector,” detecting a timing relationship between activation of the presynaptic and postsynaptic cells. In addition, because of the intrinsic kinetics of ligand gating, the current through the NMDA receptor rises and decays with a much slower time course than the AMPA receptor current. As a result, the NMDA receptors contribute to a late, slow phase of the EPSC and EPSP.
Figure 10-6 The contributions of the AMPA and NMDA glutamate receptor-channels to the excitatory postsynaptic current. These voltage-clamp current records are from a cell in the hippocampus. Similar receptor-channels are present in motor neurons and throughout the brain. (Adapted, with permission, from Hestrin et al. 1990.)
A. The drug APV selectively binds to and blocks the NMDA receptor. Shown here is the excitatory postsynaptic current (EPSC) before and during application of 50 µM APV at three different membrane potentials. The difference between the traces (blue region) represents the contribution of the NMDA receptor-channel to the EPSC. The current that remains in the presence of APV is the contribution of the AMPA receptor-channels. At -80 mV there is no current through the NMDA receptor-channels because of pronounced Mg2+ block (see Figure 10-5). At -40 mV a small late inward current through NMDA receptor-channels is evident. At +20 mV the late component is more prominent and has reversed to become an outward current. The vertical dotted line indicates the time 25 ms after the peak of the synaptic current, which is used for the calculations of late current in part B.
B. The postsynaptic currents through the NMDA and AMPA receptor-channels differ in their dependence on the membrane potential. The current through the AMPA receptor-channels contributes to the early phase of the synaptic current (filled triangles). The early phase is measured at the peak of the synaptic current and plotted here as a function of membrane potential. The current through the NMDA receptor-channels contributes to the late phase of the synaptic current (filled circles). The late phase is measured 25 ms after the peak of the synaptic current (dotted line in part A), a time at which the AMPA receptor component has decayed almost to zero. Note that the AMPA receptor-channels behave as simple resistors; current and voltage have a linear relationship. In contrast, current through the NMDA receptor-channels is nonlinear and increases as the membrane is depolarized from -80 to -40 mV, owing to progressive relief of Mg2+ block. The reversal potential of both receptor-channel types is at 0 mV. The components of the synaptic current in the presence of 50 µm APV are indicated by the unfilled circles and triangles. Note how APV blocks the late (NMDA receptor) component but not the early (AMPA receptor) component of the EPSC.
As most glutamatergic synapses contain AMPA receptors that are capable of triggering an action potential, what is the function of the NMDA receptor? At first glance the function of these receptors is even more puzzling because they are normally blocked by Mg2+ at the resting potential. However, when glutamate is paired with depolarization, the NMDA receptors uniquely conduct Ca2+ into the postsynaptic cell. This leads to a rise in intracellular [Ca2+] that can activate various calcium-dependent signaling cascades, including calcium-calmodulin-dependent protein kinase II (CaMKII) (see Chapter 11). Thus NMDA receptor activation can translate electrical signals into biochemical ones. Some of these biochemical reactions lead to long-lasting changes in synaptic strength, a set of processes called long-term synaptic plasticity that are thought to be important during synapse development and for regulating neural circuits in the adult brain. In particular, an NMDA receptor-dependent long-term potentiation (LTP) of excitatory synaptic transmission has been implicated in certain forms of memory storage (see Chapters 66 and 67).
However, there is also a potential downside to the entry of Ca2+ through the NMDA receptors. Excessively high concentrations of glutamate are thought to result in an overload of Ca2+ in the postsynaptic neurons. Such high levels of Ca2+ can be toxic to neurons. In tissue culture even a brief exposure to high concentrations of glutamate can kill many neurons, an action called glutamate excitotoxicity. The high concentrations of intracellular Ca2+ are thought to activate calcium-dependent proteases and phospholipases and lead to the production of free radicals that are toxic to the cell. Glutamate toxicity may contribute to cell damage after stroke, to the cell death that occurs with episodes of rapidly repeated seizures experienced by patients who have status epilepticus, and to degenerative diseases such as Huntington disease. Agents that selectively block the NMDA receptor may protect against the toxic effects of glutamate and have been tested clinically. Unfortunately, the hallucinations that accompany NMDA receptor blockade have so far limited the usefulness of such compounds. A further complication of attempts to control excitotoxicity by blocking NMDA receptor function is that physiological levels of NMDA receptor activation can actually protect neurons from damage and cell death.
The Excitatory Ionotropic Glutamate Receptors Are Encoded by a Distinct Gene Family
What are the molecular bases for the biophysical function of glutamate receptors and how are these receptors related to other ligand-gated ion channels? Over the past 20 years the genes coding for the subunits of all the major neurotransmitter receptors have been identified. This molecular analysis demonstrates evolutionary linkages among the structure of receptors that enable us to classify them into three distinct families (Figure 10–7). One family includes the genes encoding the kainate, AMPA, and NMDA receptors; the genes encoding the AMPA and kainate receptors are more closely related to one another than are the genes encoding the NMDA receptors. Surprisingly this gene family bears little resemblance to the two other gene families that encode ionotropic receptors (one that encodes the ACh, GABA, and glycine receptors, and one that encodes ATP receptors, as described below).
Figure 10-7 The three families of ligand-gated channels.
A. The nicotinic ACh, GABAA, and glycine receptor-channels are all pentamers composed of several types of related subunits. As shown here, the ligand-binding domain is formed by the extracellular amino-terminal region of the protein. Each subunit has a membrane domain with four membrane-spanning α-helixes (M1–M4) and a short extracellular carboxyl terminus. The M2 helix lines the channel pore.
B. The glutamate receptor-channels are tetramers, often composed of two different types of closely related subunits (here denoted 1 and 2). The subunits have a large extracellular amino terminus, a membrane domain with three membrane-spanning α-helixes (M1, M3, and M4), a large extracellular loop connecting the M3 and M4 helixes, and an intracellular carboxyl terminus. The M2 segment forms a loop that dips into and out of the cytoplasmic side of the membrane, contributing to the selectivity filter of the channel. The glutamate binding site is formed by residues in the extracellular amino terminus and in the M3-M4 extracellular loop.
C. The ATP receptor-channels (or purinergic P2X receptors) are trimers. Each subunit possesses two membrane-spanning α-helixes (M1 and M2) and a large extracellular loop that binds ATP. The M2 helix lines the pore.
Unlike the pentameric nicotinic ACh receptor family, the AMPA, kainate, and NMDA receptors are tetrameric proteins with four subunits arranged around a central pore. The AMPA receptor subunits are encoded by four separate genes (GluA1-GluA4), and there are five different kainate receptor subunit genes (GluK1-GluK5). Most of the AMPA and kainate receptors are heteromers composed of two different types of GluA and GluK subunits, respectively. The NMDA receptors are encoded by a family consisting of five genes that fall into two groups, the single GluN1 gene and the four GluN2A-D genes. Each NMDA receptor contains two GluN1 subunits and two of the different types of GluN2 subunits.1 In addition, many of these subunit genes are alternatively spliced, generating further diversity. Autoantibodies to the AMPA receptor GluA3 subunit are thought to play an important role in some forms of epilepsy. These antibodies actually mimic glutamate by activating GluA3-containing receptors, resulting in excessive excitation and seizures.
The amino acid sequence of the ionotropic glutamate receptor subunits and subsequent functional and biochemical studies provided the initial compelling evidence that the transmembrane topology of these subunits is very different from that of the nicotinic ACh receptor (Figure 10–7). Our understanding of the ionotropic glutamate receptors was then greatly expanded by Eric Gouaux and colleagues’ determination of the high-resolution X-ray crystal structures of the isolated AMPA receptor ligand binding domain and of an intact AMPA receptor-channel formed by GluA2 subunits (Figure 10–8).
Figure 10-8 Structure of an ionotropic glutamate receptor.
A. Schematic organization of the ionotropic glutamate receptors. The receptors contain a large extracellular amino terminus, followed by a transmembrane domain containing three membrane-spanning α-helixes (M1, M3, and M4) and a loop that dips into the cytoplasmic side of the membrane (M2). The ligand-binding domain is formed by the extracellular region of the receptor on the amino-terminal side of the M1 segment and by the extracellular loop connecting M3 and M4. These two regions intertwine to form a clamshell structure that binds glutamate and various pharmacological agonists and competitive antagonists. A second clamshell structure is formed at the extreme amino terminus of the receptor. This amino-terminal domain is thought to modulate receptor function and synapse development. It does not bind glutamate in the ionotropic receptors. (Reproduced, with permission, from Armstrong et al. 1998.)
B. Three-dimensional X-ray crystal structure of an AMPA receptor composed solely of GluA2 subunits. A side view of the structure of a single GluA2 subunit showing the amino-terminal domain, ligand-binding domain, and transmembrane domain. The M1, M3, and M4 transmembrane α-helixes are indicated, as is the short α-helix in the M2 loop. A molecule of a competitive antagonist of glutamate bound to the ligand-binding domain is shown in a space-filling representation. The cytoplasmic loops connecting the membrane α-helixes were not resolved in the structure and have been drawn as dashed lines. (Reproduced, with permission, from Sobolevsky, Rosconi and Gouaux, 2009.)
C. A side view of the structure of the tetrameric receptor. The four GluA2 subunits associate through the extracellular domains as a pair of dimers (two-fold symmetry). In the amino-terminal domain, one dimer is formed by the blue and yellow subunits, and the other dimer is formed by the red and green subunits. In the ligand-binding domain, the subunits change partners. In one dimer the blue subunit associates with the red subunit, whereas in the other dimer the yellow subunit associates with the green subunit. In the transmembrane region the subunits associate as a four-fold symmetric tetramer. This is a highly unusual subunit arrangement whose significance is not fully understood. (Reproduced, with permission, from Sobolevsky, Rosconi and Gouaux, 2009.)
Glutamate Receptors Are Constructed from a Set of Modules
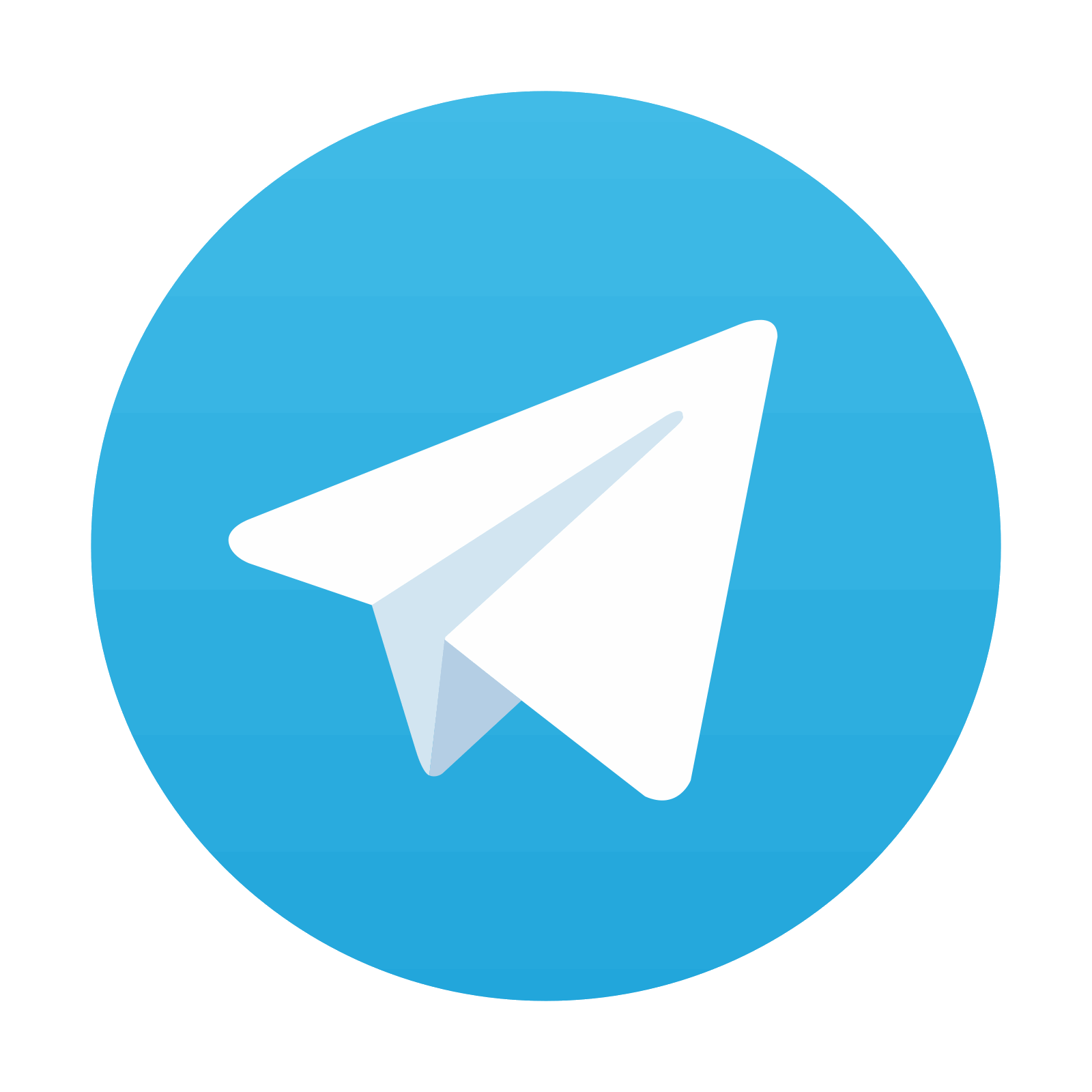
Stay updated, free articles. Join our Telegram channel
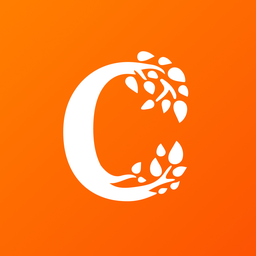
Full access? Get Clinical Tree
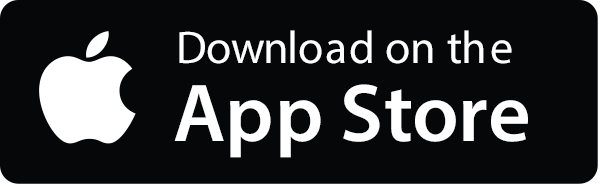
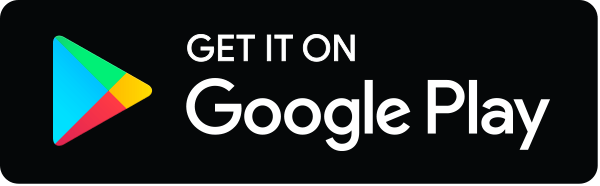