The Auditory Central Nervous System
Multiple Types of Information Are Present in Sounds
The Neural Representation of Sound Begins in the Cochlear Nuclei
The Medial Superior Olive Generates a Map of Interaural Time Differences
The Lateral Superior Olive Detects Interaural Intensity Differences
Efferent Signals from the Superior Olivary Complex Provide Feedback to the Cochlea
Midbrain Sound-Localization Pathways Are Sensitive to Experience in Early Life
The Inferior Colliculus Transmits Auditory Information to the Cerebral Cortex
Auditory Information Is Processed in Multiple Cortical Areas
Insectivorous Bats Have Cortical Areas Specialized for Behaviorally Relevant Features of Sound
Auditory Circuits in the Cerebral Cortex Are Segregated into Separate Processing Streams
The Cerebral Cortex Modulates Processing in Subcortical Auditory Areas
Hearing Is Crucial for Vocal Learning and Production in Both Humans and Songbirds
Both Humans and Songbirds Possess Specialized Neural Networks for Vocalization
BECAUSE OF ITS ROLE IN THE UNDERSTANDING and production of speech, auditory perception is one of the most important sensory modalities in humans. In most animals hearing is crucial for localizing and identifying sounds; for some species, hearing additionally guides the learning of vocal behavior.
Once sounds have been transformed into electrical responses in the cochlea, a rich hierarchy of auditory circuits analyzes and processes these signals to give rise to auditory perception. The auditory system differs from most other sensory systems in that the location of stimuli in space is not conveyed by the spatial arrangement of the afferent pathways. Instead, the localization and identification of sounds is constructed from patterns of frequencies mapped at the two ears as well as from their relative intensity and timing. The auditory system is also notable for its temporal sensitivity; time differences as small as 10 μs can be detected. Auditory pathways resemble other sensory systems, however, in that different features of acoustic information are processed in discrete circuits that eventually converge to form complex representations of sound.
In addition to studies of primates and mammals such as cats and rodents, research on animals with especially acute or specialized auditory capacities—frogs, bats, barn owls, and songbirds—has provided a wealth of information about auditory processing. Many of the principles learned from the study of such auditory specialists have proven to be generally applicable. They are simply easier to detect in animals with specialized mechanisms.
Multiple Types of Information Are Present in Sounds
Hearing helps to alert animals to the presence of unseen dangers or opportunities, and in many species also serves as a basis for communication. Information about where sounds arise and what they mean must be extracted from the representations of the physical characteristics of sound at each of the ears. To understand how animals process sound, it is useful first to consider what cues are available.
Most vertebrates take advantage of having two ears for localizing sounds in the horizontal plane. Sound sources at different positions in that plane affect the two ears differentially: Sound arrives earlier and is more intense at the ear nearer the source (Figure 31–1A). The size of the head determines how interaural time delays are related to the location of sound sources; the neuronal circuitry determines the precision with which time delays are resolved. Because sound travels at roughly 340 m/s in air, the maximal interaural delay in humans is approximately 600 μs; in small birds the greatest delay is only 35 μs. Humans can resolve the location of a sound source directly ahead to within approximately 1 degree, corresponding to an interaural time difference of 10 μs. Interaural time differences are particularly well conveyed by neurons that encode relatively low frequencies, for groups of these neurons can fire at the same position in every cycle of the sound and in this way encode the interaural time difference as an interaural phase difference.
Figure 31-1 Cues for localizing sound sources.
A. A sound arising in the horizontal plane arrives differently at the two ears. Sounds arrive earlier and are louder at the ear nearer the source. Interaural time and intensity differences are cues for localizing sound sources in the horizontal plane, or azimuth. A sound that arises directly in the front or back travels the same distance to the right and left ears and thus arrives at both ears simultaneously. A sound that arises from the side travels a shorter distance to the near ear than to the far ear and thus arrives at the near ear before it arrives at the far ear. In humans the maximal interaural time difference is approximately 600 μs. High-frequency sounds, with short wavelengths, are deflected by the head, producing a sound shadow on the far side. Interaural intensity differences are used by mammals as an additional cue for localizing sounds in the horizontal plane. Interaural time and intensity do not vary with the movement of sound sources in the vertical plane, so it is impossible to localize a pure sinusoidal tone in the vertical plane. (Adapted, with permission, from Geisler 1998.)
B. Mammals can localize broadband sounds in both the vertical and horizontal planes on the basis of spectral filtering. When a noise that has equal energy at all frequencies over the human hearing range (white noise) is presented through a speaker, the ear, head, and shoulders cancel energy at some frequencies and enhance others. The amount of sound energy at each frequency at the ear canal is shown by the traces beside each speaker, which plot in decibels the power spectrum of sound that reaches the eardrum relative to the white noise that is produced by the speakers. For a white noise the power spectrum is flat. Note that by the time the noise has reached the bottom of the ear canal its spectrum is no longer flat. The small plot in the upper right compares spectral filtering of sounds coming from low in the front (blue) with sounds coming from behind and above the listener’s head (brown). At high frequencies filtering by the ear introduces deep notches into spectra that vary depending on where the sounds arose. Sounds that lack energy at high frequencies and narrowband sounds are difficult to localize in the vertical plane. Spectral filtering also varies in the horizontal plane and provides the only location cue to animals that have lost hearing in one ear. You can test the salience of these spectral cues with a simple experiment. Close your eyes as a friend jingles keys directly in front of you at various elevations. Compare your ability to localize sounds under normal conditions and when you distort the shape of both ears by pushing them with your fingers from the back. The numerical values of the sound waves are measured in degrees beginning in front of the listener(–60 degrees) and moving in 20-degree increments in a vertical arc until the sound is broadcast behind the listener at 240 degrees. (Data reproduced, with permission, from D. Kistler and F. Wightman.)
Sounds of high frequencies produce sound shadows or intensity differences between the two ears. For many mammals with small heads, high-frequency sounds provide the primary cue for localizing sound in the horizontal plane.
Spectral filtering in mammals allows sounds to be localized in the vertical plane and with a single ear (Figure 31–1B). High-frequency sounds, with wavelengths that are close to or smaller than the dimensions of the head, shoulders, and external ears, interact with those parts of the body to produce constructive and destructive interference, introducing broad spectral peaks and narrow, deep spectral notches whose frequency changes with the location of the sound. High-frequency sounds from different origins are filtered differently because in mammals the shape of the external ear differs back-to-front as well as top-to-bottom. Animals learn to use these spectral cues to locate sound sources. If the shape of the ear is experimentally altered, even adult humans can learn to make use of a new pattern of spectral cues. If animals lose hearing in one ear, they lose interaural timing and intensity cues and must depend completely on spectral cues for localizing sounds.
How do we make sense of the sounds that we hear? Most natural sounds contain energy over a wide range of frequencies and change rapidly over time. The information used to recognize sounds varies among animal species, and depends on listening conditions and experience. Human speech, for example, can be understood in the midst of noise, over electronic devices that distort sounds, and even through cochlear implants. One reason for its robustness is that speech contains redundant cues: The vocal apparatus produces sounds in which multiple parameters covary. At the same time, this makes the task of understanding how animals recognize patterns a complicated one. It is not clear which cues are used by animals under what conditions.
Music is a source of pleasure to human beings. Musical instruments and human voices produce sounds that have energy at the fundamental frequency that corresponds to the perceived pitch as well as at multiples of that frequency that give sounds a quality that allows us, for example, to distinguish a flute from a violin when their pitch is the same. Musical pitches are largely in the low frequency range in which auditory nerve fibers fire in phase with sounds. Musical sounds when combined simultaneously produce chords, and chord progressions produce melodies. Euphonious, pleasant chords elicit regular, periodic firing in the auditory nerve in which the most common interval between action potentials corresponds to the period of the perceived pitch. In dissonant sounds there is less regularity both in the sound itself and in the firing of auditory nerve fibers; the component frequencies are so close that they interfere with one another instead of periodically reinforcing one another.
The Neural Representation of Sound Begins in the Cochlear Nuclei
The neural pathways that process acoustic information extend from the ear to the brain stem, through the midbrain and thalamus, to the cerebral cortex (Figure 31–2). Acoustic information is conveyed from the cochlea by the central processes of cochlear ganglion cells (see Figure 30–17) that terminate in the cochlear nuclei in the brain stem. There information is relayed to several different types of neurons, most of which are arrayed tonotopically.
Figure 31-2 The central auditory pathways extend from the brain stem through the midbrain and thalamus to the auditory cortex. All cochlear (eighth cranial) nerve fibers terminate in the cochlear nuclei of the brain stem. The neurons of these nuclei project in several parallel pathways to the inferior colliculus. Their axons exit through the trapezoid body, intermediate acoustic stria, or dorsal acoustic stria. Some cells terminate directly in the inferior colliculus. Others contact cells in the superior olivary complex and in the nuclei of the lateral lemniscus, which in turn project to the inferior colliculus. Neurons of the inferior colliculus project to the superior colliculus and to the medial geniculate nucleus of the thalamus. Thalamic neurons project to the auditory cortex. Only the cochlear nuclei and the ventral nuclei of the lateral lemniscus receive monaural input. (Adapted, with permission, from Brodal 1981.)
The axons of each of these types of neurons take a different route and terminate on separate targets in the brain stem and midbrain, ultimately bringing acoustic information mainly to the contralateral inferior colliculus. Some of the pathways from the cochlear nuclei to the inferior colliculus are direct; others involve one or two synaptic stages in brain stem auditory nuclei. From the inferior colliculi acoustic information flows two ways: to the ipsilateral superior colliculus, where it participates in orienting the head and eyes in response to sounds, and to the ipsilateral thalamus, the relay to auditory areas of the cerebral cortex. The afferent auditory pathways from the periphery to higher brain regions include efferent feedback at many levels.
The Cochlear Nerve Imposes a Tonotopic Organization on the Cochlear Nuclei and Distributes Acoustic Information into Parallel Pathways
The afferent nerve fibers from cochlear ganglion cells are bundled in the cochlear or auditory component of the vestibulocochlear (eighth cranial) nerve and terminate exclusively in the cochlear nuclei. The cochlear nerve in mammals contains two groups of fibers: a large contingent (95%) of myelinated fibers that receives input from inner hair cells, and a small number (5%) of unmyelinated fibers that receives input from outer hair cells.
The larger, more numerous, myelinated fibers are much better understood than the unmyelinated fibers. Each myelinated fiber detects energy over a narrow range of frequencies; together these fibers carry information about the moment-to-monent variation in the frequency content of sounds. The unmyelinated fibers terminate on the large neurons in the ventral cochlear nuclei and on the small granule cells that surround the ventral cochlear nuclei. These fibers integrate information from a relatively wide region of the cochlea and are therefore unlikely to be as sharply tuned as the myelinated fibers. Because it is difficult to record from these tiny fibers, the information they convey to the brain is unknown. Indirect evidence suggests that they encode the intensity of sounds over a wide dynamic range.
Two features of the cochlear nuclei are important. First, the cochlear nerve fibers terminate in these nuclei in a tonotopic organization. Fibers that carry information from the apical end of the cochlea, which detects low frequencies, terminate ventrally in the ventral and dorsal cochlear nuclei; those that carry information from the basal end of the cochlea, which detects high frequencies, terminate dorsally (Figure 31–3). Second, each cochlear nerve fiber innervates several different areas within the cochlear nuclei, contacting various types of neurons that have distinct projection patterns to higher auditory centers. As a result, the auditory pathway is split into at least four parallel ascending pathways that simultaneously extract different facets of acoustic information from the representation of sound carried by cochlear nerve fibers.
Figure 31-3 Cochlear nerve fibers terminate in the dorsal and ventral cochlear nuclei in a tonotopic organization.
A. Stimulation with three frequencies of sound causes the basilar membrane (uncoiled for illustration) to vibrate at three positions, exciting distinct populations of hair cells and their afferent nerve fibers.
B. Cochlear nerve fibers project in a tonotopic pattern to the cochlear nuclei. Those encoding the lowest frequencies terminate most ventrally, whereas those encoding higher frequencies terminate more dorsally. The cochlear nuclei include the unlayered ventral cochlear nucleus and the layered dorsal cochlear nucleus. Each afferent fiber enters at the nerve root and splits into branches that run anteriorly (the ascending branch) and posteriorly (the descending branch). The ventral cochlear nucleus is thus divided functionally into anteroventral and posteroventral divisions. The orderly termination of cochlear nerve fibers imposes a tonotopic map on each subdivision of the ventral cochlear nucleus and on the dorsal cochlear nucleus.
The Ventral Cochlear Nucleus Extracts Information About the Temporal and Spectral Structure of Sounds
The principal cells of the unlayered ventral cochlear nucleus sharpen timing and spectral information and convey it to other auditory nuclei in the brain stem. Three types of neurons are intermingled and form separate pathways through the brain stem.
Bushy cells project bilaterally to the superior olivary complex. This pathway has two parts, one through the medial superior olive and the other through the lateral superior olive and medial nucleus of the trapezoid body. Large spherical bushy cells sense low frequencies and project bilaterally to the medial superior olive, forming a circuit that detects interaural time delay and permits the localization of low-frequency sounds in the horizontal plane. Small spherical bushy cells and globular bushy cells sense high frequencies and are associated with the lateral superior olive. Small spherical bushy cells probably excite the lateral superior olive ipsilaterally. The globular bushy cells, through calyceal axonal endings that surround the postsynaptic neurons, excite neurons in the contralateral medial nucleus of the trapezoid body that in turn inhibit principal cells of the lateral superior olive. The pathways through the lateral superior olive are involved in the detection of interaural intensity differences and contribute to the localization of high-frequency sounds in the horizontal plane.
Stellate cells excite neurons in the ipsilateral dorsal cochlear nucleus, probably in the ipsilateral lateral superior olive, in the periolivary nuclei, and in the contralateral ventral nucleus of the lateral lemniscus through collaterals of axons that project to the contralateral inferior colliculus. The tonotopic array of stellate cells encodes the spectra of sounds.
Octopus cells excite targets in the contralateral periolivary region and the ventral nucleus of the lateral lemniscus. These neurons detect onset transients and periodicity in sounds and may be involved in the recognition of sound patterns.
The differences in the integrative tasks performed by these pathways are evident in the synaptic structures and shapes of the three types of neurons. The shapes of their dendrites differ, reflecting differences in the way they collect information from cochlear nerve fibers (Figure 31–4A,B). The dendrites of bushy and stellate cells span only a small range of the tonotopic array of auditory nerve fibers, receive input from relatively few auditory nerve fibers, and are consequently sharply tuned. Many of the inputs to bushy cells are from unusually large terminals that surround the cell bodies, meeting the need for large synaptic currents. Octopus cells, in contrast, have dendrites that span a large proportion of the array of afferent fibers, receiving input from many cochlear nerve fibers, and thus are broadly tuned. Their need for large synaptic currents is met by summing inputs from large numbers of small terminals.
Figure 31-4 (Opposite) Cells in the cochlear nuclei extract acoustic information from the distinct electrophysiological properties of individual cochlear nerve fibers.
A. The terminals along the length of each cochlear nerve fiber in the ventral cochlear nucleus differ in size and shape, reflecting differences in their targets. Large end bulbs form synapses on bushy cells; smaller boutons contact stellate and octopus cells. The nerve fibers are color-coded as in Figure 30–3: The yellow fiber encodes the highest frequencies and the red fiber the lowest. (Adapted, with permission, from Cajal 1909.)
B. A layer of granule cells separates the unlayered ventral cochlear nucleus (beige) from the layered dorsal nucleus (brown). In the dorsal cochlear nucleus the cell bodies of fusiform and granule cells are intermingled in a region between the outermost molecular layer and the deep layer. Cochlear nerve fibers, color-coded for frequency as in part A, terminate in both nuclei but with different patterns of convergence on the principal cells. Bushy, stellate, and fusiform cells each receive input from a few auditory nerve fibers and are sharply tuned, whereas individual octopus cells are contacted by many auditory nerve fibers and are broadly tuned.
C. Differences in the intrinsic electrical properties of the principal cells of the cochlear nuclei are reflected in the patterns of voltage change in the cells. When steadily depolarized, stellate and fusiform cells fire repetitive action potentials, whereas low-voltage-activated conductances prevent repetitive firing in bushy and octopus cells. The low input resistance of bushy and octopus cells in the depolarizing voltage range makes voltage changes rapid but also small; the rise and fall of voltage changes in stellate and fusiform cells is slower. Synaptic currents, too, produce different synaptic potentials. The synaptic potentials are brief in octopus and bushy cells but longer-lasting in stellate and fusiform cells. The brief synaptic responses in bushy and octopus cells require larger synaptic currents but encode the timing of auditory nerve inputs more faithfully than do the longer-lasting responses of stellate or fusiform cells. (Reproduced, with permission, from N. Golding.)
The biophysical properties of neurons determine how synaptic currents are converted to voltage changes and over how long a time synaptic inputs are integrated. Octopus and bushy cells in the ventral cochlear nucleus are able to respond with exceptionally rapid and precisely timed synaptic potentials. These neurons have a prominent, low-voltage-activated K+ conductance that confers a low input resistance and rapid responsiveness and prevents repetitive firing (Figure 31–4C). The large synaptic currents that are required to trigger action potentials in these leaky cells are delivered through rapidly gated, high-conductance, AMPA-type (α-amino-3-hydroxy-5-methylisoxazole-4-propionate) glutamate receptors found at many synaptic release sites. In contrast, stellate cells, in which even relatively small depolarizing currents produce large, protracted voltage changes, generate slower excitatory postsynaptic potentials (EPSPs) in response to synaptic currents. NMDA-type (N-methyl-D-aspartate) glutamate receptors enhance the slow responses.
Neurons in the ventral cochlear nucleus are able to encode different features of sounds because of differences in the pattern of input and their biophysical properties. Octopus cells detect synchronous firing in cochlear nerve fibers with exceptional temporal precision. Large, low-voltage-activated K+, and hyperpolarization-activated conductances give octopus cells exceptionally low input resistances so that individual cochlear nerve fibers can produce synaptic responses of only approximately 1 mV and only 1 ms in duration. To fire action potentials octopus cells require summation of the rising phases of numerous synaptic inputs. Individual octopus cells detect coincident firing in the relatively large number of cochlear nerve fibers (more than 60) that contact them. Coincident firing in large numbers of cochlear nerve fibers is produced by periodic sounds such as vowels and musical sounds and by the onset of broadband sounds found in consonants or clicks.
Compared to octopus cells, bushy cells convey a more sharply tuned but less temporally precise version of the firing patterns of cochlear nerve fibers. The roughly 10 cochlear nerve fibers that terminate on each bushy cell deliver relatively large synaptic currents that require summation of only a few inputs to trigger an action potential. Two properties of bushy cells enable these neurons to encode with precision the detailed temporal structure of sounds. The cells’ low input resistance shortens the voltage changes produced by the incoming synaptic currents; their need to summate several inputs removes variability in the timing of firing in cochlear nerve fibers by averaging.
The temporal fine structure of sounds that bushy cells encode provides information about the relative time of arrival of inputs to the two ears and is used at the next synaptic stage to form a map of the interaural time differences that underlie the ability to localize sound sources in the horizontal plane. The detection of musical pitch also requires the encoding of the temporal fine structure of sounds, but whether that information is carried through octopus or bushy cells or through a combination of pathways is not known.
Individual stellate cells detect intensity over a narrow frequency range and as a population they provide a continuous representation of the spectrum of sound energy. They are sharply tuned, being driven by only approximately 10 cochlear nerve fibers. Feedforward excitation through other, similarly tuned stellate cells and enhancement of inputs through NMDA-type receptors compensates for adaptation and obscures the fine structure of sound, encoded by cochlear nerve inputs, but allows the tonic firing rate of the cells to reflect the intensity of sounds to which they are tuned. Sideband inhibition enhances the cells’ encoding of spectral peaks and troughs. Stellate cells track modulations in intensity on a timescale of tens of milliseconds that is known to be important for understanding speech.
The Dorsal Cochlear Nucleus Integrates Acoustic with Somatosensory Information in Making Use of Spectral Cues for Localizing Sounds
In mammals cochlear nerve fibers extend into a layered dorsal cochlear nucleus (Figure 31–4A,B). The dorsal cochlear nucleus receives input from two systems of neurons that project to different layers.
The outermost molecular layer is the terminus of a system of parallel fibers, the unmyelinated axons of granule cells that are scattered in and around the cochlear nuclei. The parallel fibers terminate on interneurons that bear a strong resemblance to those in the cerebellum. This system transmits somatosensory, vestibular, and auditory information from widespread regions of the brain to the molecular layer.
The deep layer is the terminus of cochlear nerve fibers, which convey acoustic information directly and indirectly through stellate cells of the ventral cochlear nucleus. The cochlear nerve inputs are tonotopically organized in isofrequency laminae that run at right angles to parallel fibers.
Inputs from both systems of fibers are combined in the principal neurons of the dorsal cochlear nucleus, the fusiform cells. Parallel fibers excite fusiform cells on their spiny apical dendrites in the molecular layer at plastic synapses, whereas cochlear nerve fibers and stellate cells of the ventral cochlear nucleus excite fusiform cells on their smooth basal dendrites in the deep layer at synapses that show little plasticity. Both fiber systems also inhibit fusiform cells through inhibitory interneurons.
Eric Young and his colleagues proposed that the dorsal cochlear nucleus helps mammals interpret spectral cues for localization of sound sources. When animals move their head or ears or walk, they affect the angle of incidence of sounds to the ears even when a sound source stays in one place. To make full use of spectral cues, animals must differentiate the predictable cues that are produced by their own movements from the more interesting, unpredictable ones that inform them about the location of external sound sources. The somatosensory and vestibular information about the position of the head and ears, as well as descending information from higher levels of the nervous system about the animal’s own movements, can serve to identify the predictable cues. Fusiform cells thus relate the spectral cues they receive through the deep layer with predictable cues received through the molecular layer.
Among vertebrates only mammals have dorsal cochlear nuclei and only mammals have been shown to make use of spectral cues. Most birds and other reptiles hear to only 5 kHz, which corresponds to a wavelength of 6.8 cm. Sounds with wavelengths that exceed 7 cm do not interact with heads whose diameters are less than 2 cm wide and thus do not provide spectral cues. Conversely, humans hear to 20 kHz, corresponding to a wavelength of 1.7 cm; cats and dogs hear to approximately 50 kHz; and mice and bats hear to 100 kHz. The wavelengths of these high-frequency sounds are small with respect to the dimensions of the heads and ears and therefore provide useful spectral cues.
The Superior Olivary Complex of Mammals Contains Separate Circuits for Detecting Interaural Time and Intensity Differences
In many vertebrates, including mammals and birds, neurons in the superior olivary complex compare the activity of cells in the bilateral cochlear nuclei to locate sound sources. Separate circuits detect interaural time and intensity differences.
The Medial Superior Olive Generates a Map of Interaural Time Differences
Differences in arrival times at the ears are not represented at the cochlea. Instead, a map of interaural phase is created in the medial superior olive by a comparison of the timing of firing in responses to sounds from the two ears. Sounds arrive at the near ear before they arrive at the far ear, with interaural time differences being directly related to the location of sound sources in the horizontal plane.
Cochlear nerve fibers tuned to frequencies below 4 kHz and their bushy cell targets encode sounds by firing in phase with the pressure waves. This property is known as phase-locking. Although individual neurons may fail to fire at some cycles, the population of neurons represents the fine structure of sound waves by firing with every cycle. In so doing, these neurons carry information about the timing of inputs with every cycle of the sound. Sounds arriving from the side evoke phase-locked firing that is consistently earlier at the near ear than at the far ear, resulting in consistent interaural phase differences (Figure 31–5A).
Figure 31-5 (Opposite) Interaural time differences localize sound sources in the horizontal plane.
A. When a sound, such as a pure tone, arises from the right, the right ear detects the sound earlier than the left ear. The difference in the time of arrival at the two ears is the interaural time delay (ITD). Cochlear nerve fibers and their bushy cell targets fire in phase with changes in sound pressure. Although individual neurons sometimes skip cycles, the population of bushy cells encodes the timing of low-frequency sounds and its frequency with every cycle. Comparison of the timing of action potentials of bushy cells at the two sides reveals the ITDs (slanted black lines).
B. Interaural time differences can be detected with delay lines. If axons have systematically differing lengths, their action potentials reach the nearest terminals before they reach the farthest ones. An array of neurons that detects coincident inputs from delay lines can produce a map of ITDs. When sounds come from the right, action potentials from the right ear must be delayed to compensate for the fact that they arrive earlier than those from the left and to allow the signals from right and left to coincide at one side of the array (mustard-colored cell). Such an arrangement of delay lines has been found in the nucleus laminaris of the barn owl, the homolog of the medial superior olivary nucleus.
C. Mammals use delay lines on the contralateral side only to form a map of interaural time differences. The bitufted neurons of the medial superior olivary nucleus form a sheet that is contacted on the lateral face by bushy cells from the ipsilateral cochlear nucleus and on the medial face by bushy cells from the contralateral cochlear nucleus. On the ipsilateral side the branches of the bushy cell axon are of equal length and thus deliver synaptic currents to their targets in the medial superior olive simultaneously. On the contralateral side the branches deliver synaptic currents first to the anterior regions, closest to the midline, and then to progressively more posterior regions. The postsynaptic neurons can detect synchronous excitation only when sounds arise from the contralateral half of space. When sounds arise from the right side, their early arrival at the right ear is compensated by progressively later arrival at neurons in the posterior region of the left medial superior olive (the mustard-colored cell is activated by a sound from the far right, as in part B). When sounds arise from the front and there is no interaural time difference, neurons in the anterior end of the medial superior olive are activated synchronously from both sides. Thus each medial superior olive forms a map of where sounds arise in the contralateral hemifield. (Adapted, with permission, from Yin 2002.)
In 1948 Lloyd Jeffress suggested that an array of detectors of coincident inputs from the two ears, transmitted through delay lines comprising axons with systematically differing lengths, could form a map of interaural time differences and thus a map of the location of sound sources (Figure 31–5B). In such a circuit conduction delays compensate for the early arrival at the near ear. Interaural time delays increase systematically as sounds move from the midline to the side, resulting in coincident firing further toward the edge of the neuronal array.
Such neuronal arrays have indeed been found in the barn owl in the homolog of the medial superior olivary nucleus. Mammals and chickens use a variant of this neuronal arrangement. The principal neurons of the medial superior olive form a sheet of one or a few cells’ thickness on each side of the midline. Each neuron has two tufts of dendrites, one extending to the lateral face of the sheet, the other projecting to the medial face of the sheet (Figure 31–5C). The dendrites at the lateral face are contacted by large spherical bushy cells from the ipsilateral cochlear nucleus, whereas the dendrites at the medial face are contacted by large spherical bushy cells of matching best frequency from the contralateral cochlear nucleus. The axons of bushy cells terminate in the contralateral medial superior olive with delay lines just as Jeffress had suggested, but the branches that innervate the ipsilateral medial superior olive are of equal length (see Figure 31–5C).
Each medial superior olive receives coincident inputs from the two ears only when sounds come from the contralateral half of space. As sound sources move from the midline to the most lateral point on the contralateral side of the head, the earlier arrival of sounds at the contralateral ear needs to be compensated by successively longer delay lines. This results in inputs from the two ears coinciding at increasingly posterior regions of the medial superior olive, thereby forming a map of interaural phase. Recent findings indicate that inhibition that is superimposed on these excitatory inputs plays a significant role in sharpening the map of interaural phase.
In responding to interaural phase, individual neurons in the medial superior olive provide ambiguous information about interaural time differences. Phase ambiguities are resolved when sounds have energy at multiple frequencies, as natural sounds almost always do. The sheet of neurons of the medial superior olive forms a representation of interaural phase along the rostrocaudal dimension but its tonotopic innervation by bushy cells imposes a tonotopic organization in the dorsoventral dimension. Sounds that contain energy at multiple frequencies evoke maximal coincident firing in a single dorsoventral column of neurons that localizes sound sources unambiguously.
Each medial superior olive thus forms a map of the location of sound sources in the contralateral hemifield. The striking difference between this spatial map and those in other sensory systems is that this map is not the result of the spatial arrangement of inputs, like retinotopic or somatosensory maps, but is inferred by the brain from computations made in the afferent pathways.
The Lateral Superior Olive Detects Interaural Intensity Differences
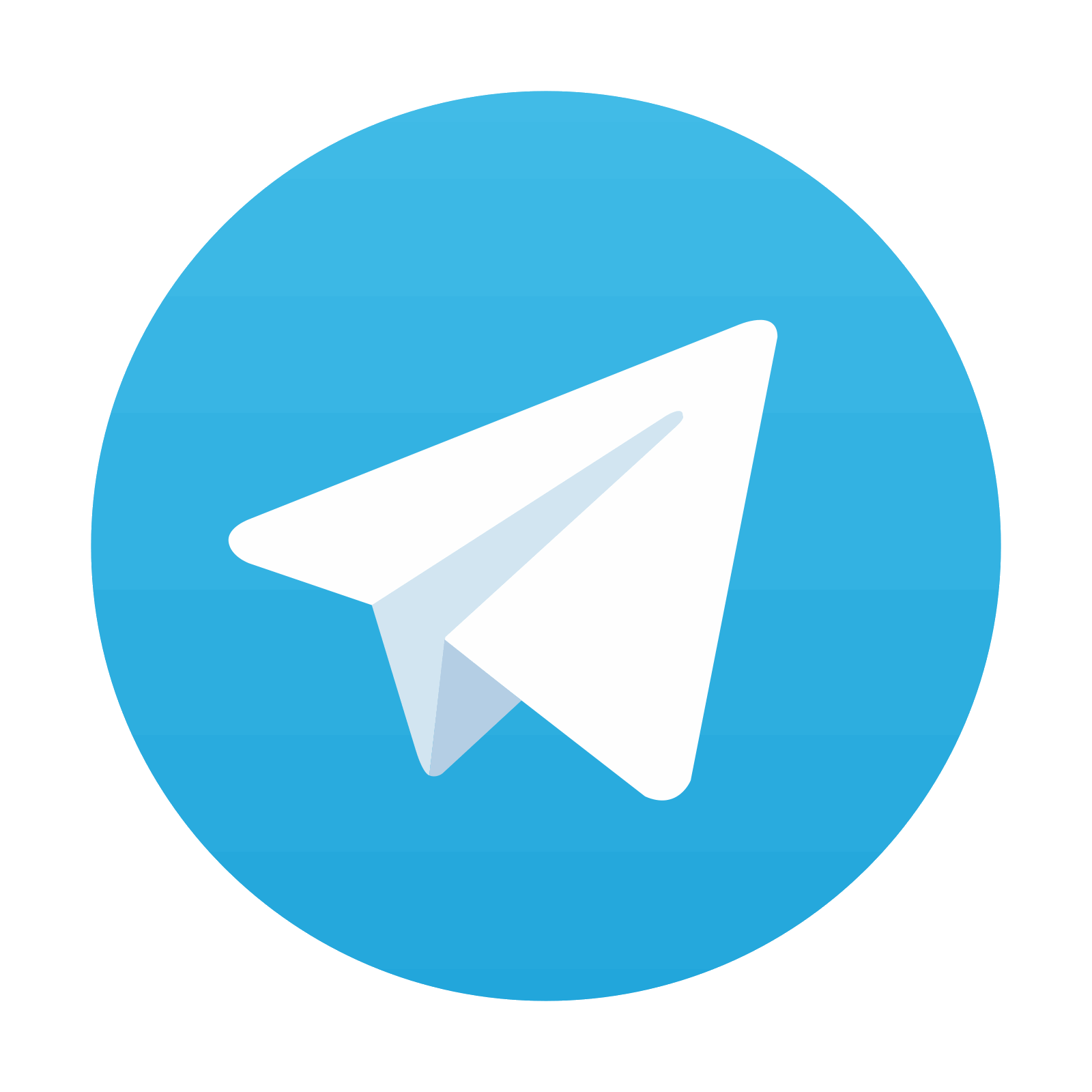
Stay updated, free articles. Join our Telegram channel
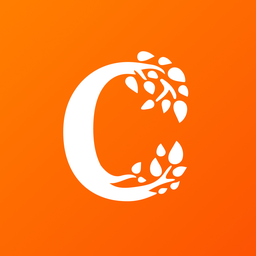
Full access? Get Clinical Tree
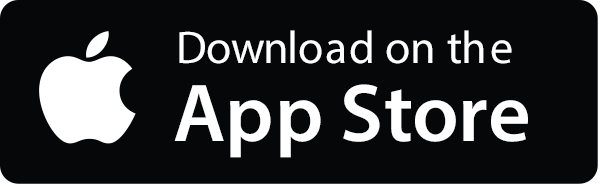
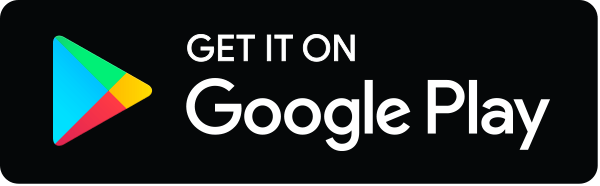