Figure 21-1. Upper panel shows the cyclic changes in a simple, pure tone. Lower panel shows that the arrival of a tone at right (D1) ear and left (D2) ear is affected by the distance traveled and shadowing effect of the head (center panel) when the source of the sound is displaced from the midline. The interaural time difference (ITD) is calculated by the equation (D2 − D1) V = ITD, where V is the speed of sound.
The normal frequency range for human hearing is 50 to 16,000 Hz. Most human speech takes place in the range of 100 to 8000 Hz, and the most sensitive part of the range is between 1000 and 3000 Hz. Exposure to loud noise can result in selective hearing loss for certain frequencies, and normal aging may reduce the range.
The hearing apparatus is exquisitely sensitive to sound intensity over an enormous dynamic range. Intensity of sound is related to the perception of loudness and is usually measured in units called decibels (dB). Intensity is also related to a measure of sound pressure level at the tympanic membrane. A sound that has 10 times the power of a just-audible sound is said to have a 20-dB sound pressure level. Normal conversational levels of sound are about 50 dB. In our industrial society, we are exposed routinely to noise in the 85- to 100-dB range, such as city traffic, lawn mowers, vacuum cleaners, and even a portable music player’s headphones. Other common examples of noises above 120 dB that may cause varying degrees of discomfort include rock concerts, thunderclaps, firecrackers, and jet engines.
The brain derives the location of a sound by computing differences in the shape, timing, and intensity of the waveforms that reach each ear. The path of the sound is affected by the distance to the ears and by obstacles such as the head (Fig. 21-1). Thus interaural time and intensity differences are related to the angle between the direction in which the head is pointing and the direction of the sound source. Interaural time differences are more important for localizing low-frequency sounds, whereas interaural intensity differences are more important for localizing high-frequency sounds.
PROCESSING OF SOUND: THE EAR
External (Outer) Ear
Sound waves are captured by the external ear (pinna) and channeled through the external auditory meatus to the tympanic membrane (Fig. 21-2A). Resonance features of the pinna and meatus enhance some frequencies more than others in a direction-dependent fashion. For example, sounds coming toward the back of the head are baffled compared with those coming toward the side of the head. Monaural (single-ear) localization depends on such cues, and accuracy in localizing sound is impaired by damage to the pinna.
Figure 21-2. A, The path of auditory signals from the external ear, through the middle ear, and into the inner ear. B, The cochlea is shown in cross section from apex to base. C, The basilar membrane functions to separate waves of different frequencies within a sound. This membrane is narrow and stiff at its base and becomes wider and more flexible toward the apex, and the hair cell stereocilia increase correspondingly in height. These features “tune” the membrane so that each frequency of sound in the audible range will cause a wave in the basilar membrane that has its peak amplitude at a unique spot (near the base for high frequencies and near the apex for low frequencies). At this spot, the hair cells are excited most intensely, producing a peak in neural output.
Middle Ear
The middle ear or tympanic cavity is an air-filled space in the temporal bone that is interposed between the tympanic membrane and the inner ear structures (Fig. 21-2A). Sounds are transmitted across the space from the tympanic membrane to the fluid-filled inner ear by a chain of three bony ossicles: the malleus, incus, and stapes. On one end of this chain, the arm of the malleus is attached to the tympanic membrane, and at the other end, the footplate of the stapes fits into the oval window of the membranous labyrinth of the inner ear. The three bones act as levers to reduce the magnitude of movements of the tympanic membrane while increasing their force at the oval window.
The mechanical stiffness of the ossicle chain acts to compensate for the difference in impedance between air and fluid environments (a function called impedance matching) so that there is optimal transfer of energy between the two media. The stiffness of the ossicle chain can also be modified by two muscles of the middle ear, the tensor tympani and stapedius muscles (middle ear reflex). Diseases such as otosclerosis and otitis media result in conductive hearing loss by affecting the efficiency of the ossicle movement. Otosclerosis, the cause of middle ear conductive hearing loss in about half of cases, may be an inherited disease and is characterized by tissue overgrowth and resultant fixation of the stapes in the oval window. Otitis media is an inflammation of the middle ear and may be accompanied by the accumulation of pus or exudate. In addition, fractures of the temporal bone with direct damage to the ossicles, or indirect damage by bleeding into the middle ear, may result in a conduction deafness.
Conduction Deafness
A conductive deafness is a deficit related to an obstructed, or altered, transformation of sound to the tympanic membrane or through the ossicle chain of the middle ear. For example, damage to the pinna results in a failure of sound waves to be properly conducted to the auditory meatus. In addition, infection involving the auditory canal (otitis externa, sometimes called swimmer’s ear), inflammation of or trauma to the tympanic membrane, and even the excessive accumulation of cerumen (wax) in the auditory canal are other causes of conduction deafness. The deficit experienced by the patient may range from decreased hearing to total deafness in the affected ear. Depending on the cause, conduction deafness may resolve with medication or by removal of the obstruction.
Inner Ear: Structure of the Cochlea
The cochlea is named for its similarity to a conch shell (Fig. 21-2B). Its main elements include the fluid-filled membranous labyrinth, specialized sensory epithelium of the organ of Corti, and neurons of the spiral ganglion with their peripheral and central axonal branches.
The membranous cochlea, the coiled portion of the inner ear, is encased in the osseous cochlea and consists of three spiraling chambers. The cochlea makes approximately two and two-thirds turns from base to apex. Uncoiled, it is about 34 mm long. The base of the cochlear spiral is connected to the saccule of the membranous labyrinth by the ductus reuniens.
The central chamber of the membranous cochlea is the cochlear duct, also called the scala media (Fig. 21-2B). Above it, the scala vestibuli is positioned to communicate with the vestibule, the portion of the membranous inner ear between the oval window and the cochlea. Below, the scala tympani ends at the round window, which separates this space from the middle ear cavity. In cross section, the scala media is bounded by the basilar membrane below, the vestibular or Reissner membrane above, and the stria vascularis externally (Figs. 21-2B and 21-3). The screw-like bony core of the cochlea is the modiolus. A spiral osseous lamina extends outward from the modiolus to join the basilar membrane. The basilar membrane, in turn, is continuous laterally with the spiral ligament. The scala vestibuli and tympani are filled with perilymph. The endolymph, which fills the cochlear duct, is elaborated by the cells and rich capillary bed of the stria vascularis (Fig. 21-3).
Figure 21-3. Cross section through a typical turn of the membranous cochlea.
The organ of Corti is the specialized sensory epithelium resting on the basilar membrane (Fig. 21-3). It is composed of inner and outer hair cells, supporting cells, and the tectorial membrane. The inner hair cells are separated from the outer hair cells by the tunnel of Corti (Fig. 21-3). This tunnel is formed by the filamentous arches of the inner and outer pillar cells and is filled with fluid.
Inner hair cells form a single line spiraling from base to apex, and the outer hair cells form three parallel lines that follow the same course (Fig. 21-4). Once damaged, human hair cells do not regenerate. Research has not yet found a way to augment this. It is uncertain how many of the inner (about 3500) or outer (about 12,000) hair cells must be lost to disease, trauma, or aging before a just-noticeable sensorineural hearing loss ensues. Projecting from the apical surface of each hair cell is a hair bundle consisting of 50 to 150 stereocilia arranged in curving rows (Fig. 21-4). Each hair bundle is polarized so that the longest stereocilia are on the outer border (Fig. 21-4), and the rows of stereocilia are linked by filamentous material at their tips.
Figure 21-4. The structure and function of the organ of Corti (lower) and the relation of type I and type II afferent fibers to the spiraling ranks of inner and outer hair cells (upper). Note that the designation lateral or medial olivocochlear efferents refers to their origin in the superior olive, not to their target in the organ of Corti.
The tectorial membrane is a gelatinous arm that extends outward over the sensory epithelium from the limbus of the osseous spiral lamina (Fig. 21-4). The taller stereocilia in each hair bundle are in contact with or embedded in the tectorial membrane. Consequently, movement of the basilar membrane and the organ of Corti will bend the stereocilia against the tectorial membrane and cause a graded depolarization of the hair cells.
The bony modiolus, around which the cochlear duct turns, houses the spiral ganglion (Figs. 21-2 and 21-3). At the edge of the osseous spiral lamina, the peripheral processes of the bipolar cells of this ganglion lose their myelin and pass through perforations to the basilar membrane, where they synapse on the base of the inner and outer hair cells (Fig. 21-4). The central processes of the spiral ganglion cells form the cochlear portion of the vestibulocochlear nerve (cranial nerve VIII). Efferent fibers to the cochlea either spiral along the inner part of the basilar membrane to synapse on inner hair cells or travel radially across the tunnel of Corti to contact outer hair cells (Fig. 21-4).
Mechanoelectrical Transduction
Inner hair cells are extremely sensitive transducers that convert the mechanical force applied to the hair bundle into an electrical signal (Fig. 21-4). Endolymph, like extracellular fluid, has a high concentration of potassium. In contrast, perilymph, like cerebrospinal fluid, has a high concentration of sodium. As indicated in Figure 21-4, the potential difference between the endolymph and the perilymph is +80 mV. This endolymphatic potential appears to be due to the selective secretion and absorption of ions by the stria vascularis. At the same time, ion pumps in the hair cell membrane produce a resting intracellular potential of about −70 mV (Fig. 21-4).
As the basilar membrane moves up in response to fluid movement in the scala tympani, the taller stereocilia are displaced against the tectorial membrane. This causes ion channels at the tips of the stereocilia to open, allowing potassium flow along the electrical gradient to depolarize the cell (Fig. 21-4). The large potential difference between the endolymph and the hair cell interior creates a force of 150 mV that drives potassium into the cell and that increases the range of the cell’s graded electrical response to mechanical displacement. Damage to the stria vascularis results in loss of the endolymphatic potential and failure of mechanoelectrical transduction.
When a hair cell depolarizes, voltage-gated calcium channels at the base of the cell open, and the resulting influx of calcium causes synaptic vesicles to fuse to the cell membrane and to release a neurotransmitter into the synaptic cleft between the hair cell and the cochlear nerve fibers (Fig. 21-4). The transmitter causes depolarization of the afferent fiber, and an action potential is transmitted along the cochlear nerve fiber.
The stimulus-related changes in the electrical potential between the perilymph and the hair cells can be recorded anywhere in the cochlea. The potential varies synchronously with the sound stimulating the ear and is therefore referred to as the cochlear microphonic. This electrical record provides a clinically useful monitor of cochlear function.
Tuning of the Cochlea
The cochlea acts as a frequency filter to separate and analyze individual frequencies from complex sounds. These tuning properties result from anatomic and physiologic characteristics of hair cells and the basilar membrane (Figs. 21-2 and 21-3).
The plunger-like motion of the stapes in the oval window compresses the perilymph. In the fluid medium of the cochlea, this pressure variation imparts motion to the basilar membrane, causing a wave to travel along it (Fig. 21-2C). The basilar membrane is stiffest at its base and becomes progressively more flexible toward its tip. Therefore any given frequency of sound (pure tone) will cause a wave in the basilar membrane that has maximum displacement that is precisely timed and spaced along the membrane. For high tones, this point is close to the base of the cochlea, and for lower frequencies, it is more distal. The response of hair cells to the tone is strongest at the point of greatest displacement. Therefore the position from base to apex along the spiral of the basilar membrane and organ of Corti is directly related to the frequency of the tone that will elicit a response. This relationship of frequency and cochlear position is the basis for the place theory of cochlear tuning. The cochleotopic order and thus tonotopic representation are highly conserved throughout the auditory pathways.
In patients with profound sensorineural hearing loss, some audible sensation may be regained with cochlear implants having a number of fine wire electrodes. Each wire is tuned to a broad frequency band from an electrical receiver, and the wires are implanted so that each stimulates nerve terminals at the appropriate tonotopic point along the cochlear spiral.
Primary Afferent Innervation and Function
The spiral ganglion is made up of two types of bipolar sensory neurons. Type I cells make up 90% to 95% of the cells in the spiral ganglion and have radial branches that synapse with only one or two inner hair cells (Fig. 21-4). As many as 20 or more type I radial fibers converge on each inner hair cell. As a result, type I cochlear nerve fibers respond to a narrow frequency range. In contrast, type II ganglion cells have widely distributed peripheral processes that traverse the tunnel of Corti and synapse with more than 10 outer hair cells (Fig. 21-4). Thus type II cochlear fibers are more sensitive to low-intensity sounds than are type I cells, but they may be less precisely tuned to frequency.
Frequency is coded in the cochlear nerve by the position of afferent fibers along the cochlear spiral. For loud sounds, each afferent fiber responds over a range of frequencies. As the intensity of the sound drops to near threshold, the frequency response range narrows. A tuning curve can be constructed that plots the threshold intensity for each frequency that will elicit a response (Fig. 21-5A). The characteristic frequency is the frequency at which the fiber has the lowest threshold. The discharge pattern of primary afferents over time to pure tone bursts is shown with poststimulus histograms of the number of action potentials summed over many presentations (Fig. 21-5B). Stimulus onset produces an initial high-frequency discharge followed by a lower sustained discharge level that is related to stimulus intensity. When the tone ends, the fiber drops back to a low, spontaneous discharge rate. For low-frequency fibers, the timing of each impulse is phase locked with the stimulus cycle, so that the fiber output preserves the timing information of the signal.
Figure 21-5. Typical response characteristics of type I cochlear afferent fibers. Frequency tuning curves (A), poststimulus time histogram of discharges through the duration of a tone burst at the characteristic frequency of a primary afferent fiber (B), and rate-intensity curve illustrating how a limited range of intensity can be coded in the response of primary afferent fibers to increasing intensity of a tone at its characteristic frequency (C).
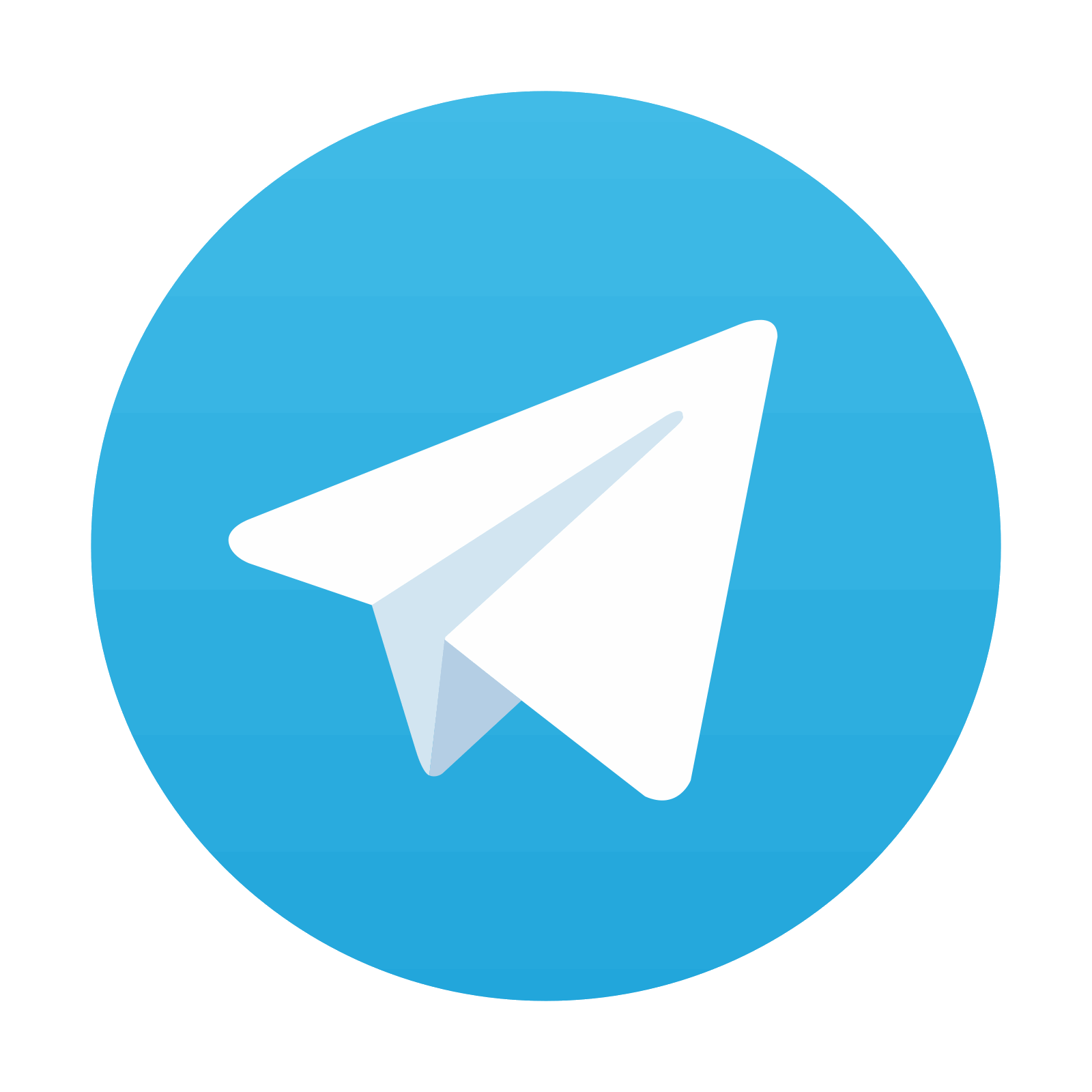
Stay updated, free articles. Join our Telegram channel
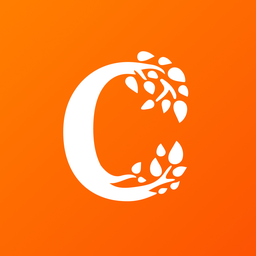
Full access? Get Clinical Tree
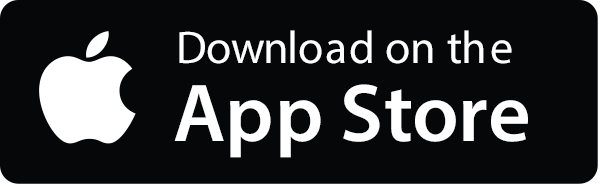
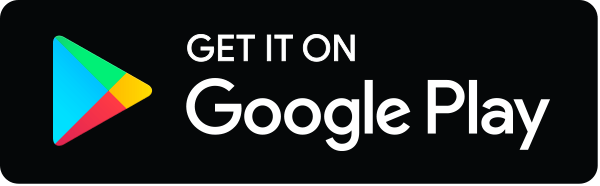