The Blood–Brain Barrier, Choroid Plexus, and Cerebrospinal Fluid
The Blood–Brain Barrier Regulates the Interstitial Fluid in the Brain
Tight Junctions Are a Major Feature of the Anatomical Blood–Brain Barrier Composition and Structure
Endothelial Enzyme Systems Form a Metabolic Blood– Brain Barrier
Brain-Derived Signals Induce Endothelial Cells to Express a Blood–Brain Barrier
Cerebrospinal Fluid Is Secreted by the Choroid Plexuses
Epithelial Cells of the Choroid Plexuses Account for the Blood–Cerebral Spinal Fluid Barrier
Brain Edema Is an Increase in Brain Volume Because of Increased Water Content
Hydrocephalus Is an Increase in Volume of Cerebral Ventricles
TO FUNCTION OPTIMALLY, NEURONS of the central nervous system and their supporting glia require a highly specialized environment. The fluids that bathe the central nervous system’s interstitial and cerebrospinal compartments are regulated by the blood–brain and blood–cerebrospinal fluid (CSF) barriers. Evidence for these barriers was first obtained in the 19th century when it was observed that acidic vital dyes stain the brain if the dye is injected into the cerebrospinal fluid but not if injected into the blood stream.
The interstitial fluid in the brain and the cerebrospinal fluid in the intraventricular and subarachnoid spaces are separately compartmentalized. Homeostasis of these fluid compartments and ultimately the intracellular compartment of brain cells is regulated to a great degree by the blood–brain and blood–CSF barriers (Figure D–1). Endothelial cells of the cerebral microvasculature form the blood–brain barrier that regulates the movement of molecules between the blood and interstitial fluid compartments. Epithelial cells of the choroid plexus produce the cerebrospinal fluid and regulate its composition.
These barriers exclude toxic substances and protect neurons from circulating neurotransmitters such as norepinephrine and glutamate, the blood levels of which can increase greatly after a meal or in response to stress. The selective exclusion by the blood–brain barrier results primarily from specialized anatomical properties of the endothelial cells that limit the passive diffusion of water-soluble substances from the blood into the interstitial and cerebrospinal fluid compartments. As a result, many metabolites required for brain growth and function must be transported selectively across the brain endothelial and choroid epithelial cell surfaces. Specific transporters deliver energy substrates, essential amino acids, and peptides to the brain and remove metabolites.
Figure D-1 Relationships between intracranial fluid compartments and the blood–brain and blood–cerebrospinal fluid (CSF) barriers. The tissue elements indicated in parentheses form the barriers. Arrows indicate the direction of fluid flow under normal conditions. (Adapted, with permission, from Carpenter 1978.)
The Blood–Brain Barrier Regulates the Interstitial Fluid in the Brain
Distinctive Properties of the Endothelial Cells of Brain Capillaries Account for the Blood–Brain Barrier
The small blood vessels of the brain are composed primarily of endothelial cells that continuously line the vessel luminal surface. On the abluminal side the endothelial cells are associated with pericytes, smooth muscle-like cells imbedded within the basement membrane of microvessels. Perivascular astrocytes extend processes that ensheathe most of the abluminal microvessel surface to form a microvessel-astroglial complex (Figure D-2). The endothelial cells and their extensive intercellular junctions are the principal component of the barrier (Figure D-3).
Figure D-2 Ultrastructural features of endothelial cells of brain capillaries and general (systemic) capillaries. The endothelial cells of brain barrier capillaries are relatively lacking in pinocytotic vesicles, contain an increased number of mitochondria to support energy-dependent transport properties, and are interconnected by very complex interendothelial tight junctions (see Figure D-5). These anatomical features in conjunction with specific transport systems (see Figure D-7) result in highly selective transport of water-soluble compounds across the barrier endothelium. Astroglial foot processes almost completely surround the brain capillaries and are thus believed to influence barrier-specific endothelial differentiation. In contrast, systemic capillaries have interendothelial clefts, fenestrae, and prominent pinocytotic vesicles. These features of systemic capillaries allow relatively nonselective diffusion across the capillary wall. (Reproduced, with permission, from Goldstein and Betz 1986.)
Figure D-3 Tight junctions between endothelial cells are the basis of the anatomical blood– brain barrier.
A. 1. When injected into arteries that supply the brain, the electron-dense tracer horseradish peroxidase is easily visualized within the brain vessel lumens (dark staining at top) but is excluded from entering the brain by interendothelial tight junctions (TJ). The luminal space appears at the top of the electron micrographs in both 1 and 2. (Reproduced, with permission, from Reese and Karnovsky 1967.) 2. When injected into the subarachnoid space, horseradish peroxidase readily diffuses between the perivascular astroglial foot processes and across the abluminal basement membrane (BM) but fails to penetrate the interendothelial tight junction. (Reproduced, with permission, from Brightman and Reese 1969.)
B. This freeze-fracture photomicrograph of isolated brain micro-vessels shows complex arrays of interendothelial tight junctions (TJ). (Reproduced, with permission, from Shivers et al. 1984.)
In capillaries of peripheral organs and in the relatively few brain capillaries that do not contribute to the barrier (eg, those of the circumventricular organs), blood-borne polar molecules diffuse across vessel walls by multiple mechanisms. This diffusion occurs through spaces between endothelial cells, through specialized cytoplasmic fenestrations, or by fluid-phase or receptor-mediated endocytosis.
Fluid-phase endocytosis is a relatively nonspecific process by which endothelial cells (and most other cells) engulf and then internalize molecules encountered in the extracellular space by vesicular endocytosis. Receptor-mediated endocytosis is a specific process in which a ligand binds to a membrane receptor on the external surface of a cell, is internalized by means of clathrin-coated vesicles, and is transported across the cell membrane. Once within the cell the vesicle may traverse the cell, fuse with the cell membrane on the opposite cell surface and release its contents to the extracellular space, or fuse with an intracellular endosome and release its contents.
Endothelial cells of blood–brain barrier vessels are relatively deficient in vesicular transport and they are also nonfenestrated. Instead they are interconnected by complex arrays of tight junctions (Figure D-3B), which block diffusion across vessel walls. All generic endothelial cells are interconnected by a modest number of tight junctional complexes and normally have low transendothelial resistance (5-10 ohm-cm2). In the vessels of the blood–brain barrier, however, extensive arrays of tight junctions generate high transendothelial resistance (2,000 ohm-cm2), excluding particles as small as K+ ions (Figure D-4).
Figure D-4 Time course of K+ entry into tissue extracellular fluid following arterial injection. The time course of K+ entry into the cerebral cortex and neck muscle is compared to the cerebral venous outflow in the sagittal sinus following bolus injection of potassium chloride (KCl) in the aortic arch.
A. In the cerebral cortex the K+ concentration remains essentially unchanged because of the blood–brain barrier to ions.
B. In the neck muscle K+ diffuses rapidly across nonbarrier vessels into the extracellular space of the muscle. (Reproduced, with permission, from Hansen et al. 1977.)
Tight Junctions Are a Major Feature of the Anatomical Blood–Brain Barrier Composition and Structure
Tight junctions of the blood–brain barrier are composed of three principal transmembranous proteins—claudins, occludins, and junctional adhesion molecules—as well as numerous cytoplasmic accessory proteins (Figure D-5).
Claudin (~ kDa) has four transmembrane domains and forms the backbone of tight junctional complexes. Of the more than 20 members of the claudin gene family, isoforms 1 and 5 are expressed at the junctions of the blood–brain barrier. Occludin (~65 kDa) is a tight junctional protein, also has four transmembrane domains, and is recruited to junctional complexes by claudins. Claudins and occludins interact intimately at the junctional membrane to influence junctional complexity and tightness. Junctional adhesion molecules (JAMs) are located lateral to the claudins and occludins and mediate cell-cell adhesive interactions required for junctional assembly. JAMs also play an important role in the transmigration of inflammatory cells across barrier endothelial cells.
Numerous accessory proteins positioned on the cytoplasmic side of tight junctions mediate interactions between the transmembrane junctional and cytoskeletal proteins and modulate dynamic junctional properties. The zona occludin proteins link the occludins and claudins to the actin cytoskeleton (Figure D-5). They also form a scaffold for other regulatory proteins such as cingulin, a myosin-like protein, and AF-6, a target of the small G protein Ras. It is currently believed that changes in protein-protein interactions at the cytoplasmic junctional–cytoskeletal interface, resulting from gene transcription and protein phosphorylation events, modulate tight junction structure and function.
Figure D-5 Protein interactions at the tight junctions between barrier endothelial cells. Occludin, claudin, and junctional adhesion molecules (JAM) are membrane-spanning junctional proteins. Claudins on adjacent endothelial cells bind homotypically and mediate interendothelial adhesive interactions. Occludins interact with claudins to enhance and modulate interendothelial permeability. JAMs localize to the periphery of junctional complexes and mediate inflammatory cell transmigration across barrier endothelial cells. The cytoplasmic accessory proteins represented by zona occludins (ZO-1, ZO-2, and ZO-3), AF-6, and 7H6, regulate the interaction of junctions with cytoskeletal components and modulate junction function via interactions with signal transduction proteins in the membrane and cytoplasm. (Adapted, with permission, from Huber et al. 2001.)
The Blood–Brain Barrier Is Permeable in Three Ways
Normal development and brain function require many substances that must cross brain microvessels. Entry into the brain is achieved primarily in three ways: (1) by diffusion of lipid soluble substances, (2) by facilitative and energy-dependent receptor-mediated transport of specific water-soluble substances, and (3) by ion channels.
Diffusion of Lipid-Soluble Substances
The brain is separated from the blood only by the large surface of endothelial cell membrane (approximately 180 cm2/g in gray matter). This membrane permits the efficient exchange of lipid-soluble gases such as oxygen (O2) and carbon dioxide (CO2), an exchange limited only by the surface area of the blood vessels and by cerebral blood flow. Barrier vessels are impermeable to molecules with poor lipid-solubility such as mannitol. The permeability coefficient of the blood–brain barrier for many substances is directly proportional to the lipid-solubility of the substance as measured by the oil–water partition coefficient (Figure D-6).
Figure D-6 The oil–water partition coefficient correlates with brain uptake of many compounds. The coefficient is an index of lipid solubility. Brain uptake of a substance is determined by comparing the extraction of the substance relative to a highly permeable tracer during a single passage through the cerebral circulation. In general, compounds with higher coefficients have a greater uptake in the brain. Uptake of the anticonvulsants phenobarbital and phenytoin is lower than predicted from their lipid-solubility partly because of their binding to plasma proteins. This explains why the onset of anticonvulsant activity from these agents is slower than that of diazepam. Uptake of glucose and L-dihydroxyphenylalanine (L-DOPA) is greater than indicated by their lipid solubility because specific carriers facilitate their transport into the brain capillary. (Reproduced, with permission, from Goldstein and Betz 1986.)
An example of the relationship of permeability and lipid-solubility is the correlation between the relative abuse potential of psychoactive drugs such as nicotine and heroin and their lipid-solubility. Increasing the lipid-solubility of drugs enhances their delivery to the brain. Drugs with great lipid-solubility, however, are poorly soluble in blood and bind to serum albumin protein, properties that reduce delivery to the brain. Lipid-solubility is not an accurate indicator of the permeability of some hydrophilic substances, such as glucose and vinca alkaloids, because of the presence of selective endothelial transport or enzyme systems that increase or inhibit permeability.
Facilitative and Energy-Dependent Transport
Most substances that cross the blood–brain barrier are not lipid-soluble and therefore enter and leave the brain by specific transport systems (Figure D-7). Because the brain uses glucose almost exclusively as its source of energy, the hexose transporter (glucose transporter isotype-1, Glut1) of the barrier endothelial cells is abundant.
Figure D-7 A complex system of polarized transporter proteins and ion channels determines the specific movement of water-soluble compounds and ions across barrier endothelial cells. Some transporters facilitate the movement of substrates down concentration gradients (eg, Glut1, LAT1, MCT1) while others actively transport substrates via energy-dependent mechanisms (eg, ATA2 and Na+-K+-ATPase). Enzyme systems such as amino acid decarboxylase (AADC) and monoamine oxidase (MAO) function as a metabolic barrier by converting within the barrier endothelial cells substances such as L-dihydroxyphenylalanine (L-DOPA) to 3,4-dihydroxphenylacetic acid (DOPAC).
Like other transporters Glut1 consists of 12-transmembrane segments. It is a facilitative, saturable, and stereospecific transporter that functions at both the luminal and abluminal endothelial cell membranes. Because it is not energy-dependent, it cannot move glucose against a concentration gradient. In fact, the net flux of glucose is driven by the higher concentration of glucose in the plasma. More than 99% of the glucose that enters barrier endothelial cells is shuttled across for use by neurons and glia.
Monocarboxylic acids such as β-hydroxybutyrate serve as the brain’s primary energy source during early development in suckling neonates and in response to starvation in mature animals. These acids are transported across the barrier endothelial cells by the monocarboxylic acid transporter-1 (MCT1).
Amino acids are transported across barrier endothelial cells primarily by three distinct carrier systems. These systems (L, A, and ASC) were initially characterized by their different patterns and mechanisms of transport, and by their preference for different amino acid analogs. The L-system preferentially transports large neutral amino acids with branched or ringed side chains such as leucine and valine. This Na+-independent, facilitative transport system is located at luminal and abluminal endothelial cell membranes. It also transports L-DOPA L-dihydroxyphenylalanine), the dopamine precursor that is the mainstay for treating Parkinson disease.
The A-system preferentially transports glycine and neutral amino acids with short linear or polar side chains, such as alanine or serine. Unlike the L-system this carrier is Na+-dependent, and pumps amino acids down a Na+ gradient that is maintained by Na+-K+-adenosine triphosphatase (ATPase), an ionic pump that uses ATP.
The ASC-system is also an energy-dependent and Na+-dependent transporter that preferentially recognizes alanine, serine, and cysteine. A-system and ASC-system expression and function are localized at the abluminal endothelial cell surface. As a consequence of this localization these carriers are the primary means of transport of small neutral amino acids out of brain up a concentration gradient.
Another transport system found to be most abundant in brain barrier microvessels belongs to a family of ATP-binding cassette transporters (ABC transporters), a large family of transmembrane proteins expressed in many cell types. The first member of this family was identified for the ability to impart multiple drug resistance (MDR) to tumor cells. The MDR transporter limits the entry into cells of a wide range of natural and synthetic hydrophobic toxins (eg, vinca alkaloids, actinomycin-D) and therapeutic compounds (eg, cyclosporin). The ability of MDR transporters to pump certain steroid hormones suggests a physiologic role. MDR transporters are expressed by barrier endothelial cells but not by endothelial cells of most other tissues. This explains why MDR substrates do not readily cross the blood–brain barrier. Mice genetically engineered to lack MDR1a gene expression are much more sensitive than wild-type controls to centrally acting toxic compounds, indicating that MDR gene expression at the blood–brain barrier protects the brain from circulating neurotoxins.
Ion Channels and Exchangers
Specific ion channels and ion transporters mediate electrolyte movement across the blood–brain barrier. A nonselective luminal ion channel that is inhibited by both amiloride and atrial natriuretic peptide has been revealed in both in vivo studies of transport across brain microvessels and patch-clamp studies of cultured brain endothelial cells. Na+/H+, and Cl−/bicarbonate exchangers also appear to function at the luminal membrane.
The abluminal membrane of brain endothelial cells has a relatively high concentration of Na+–K+– ATPase that pumps K+ from the interstitial fluid into the endothelial cell and pumps Na+ out of the cell. This ion exchange uses ATP energy. In conjunction with K+ channels in astrocytes, this abluminal endothelial pump may play an important role in removing extracellular K+ released during neuronal activity (see Chapter 6).
Endothelial Enzyme Systems Form a Metabolic Blood–Brain Barrier
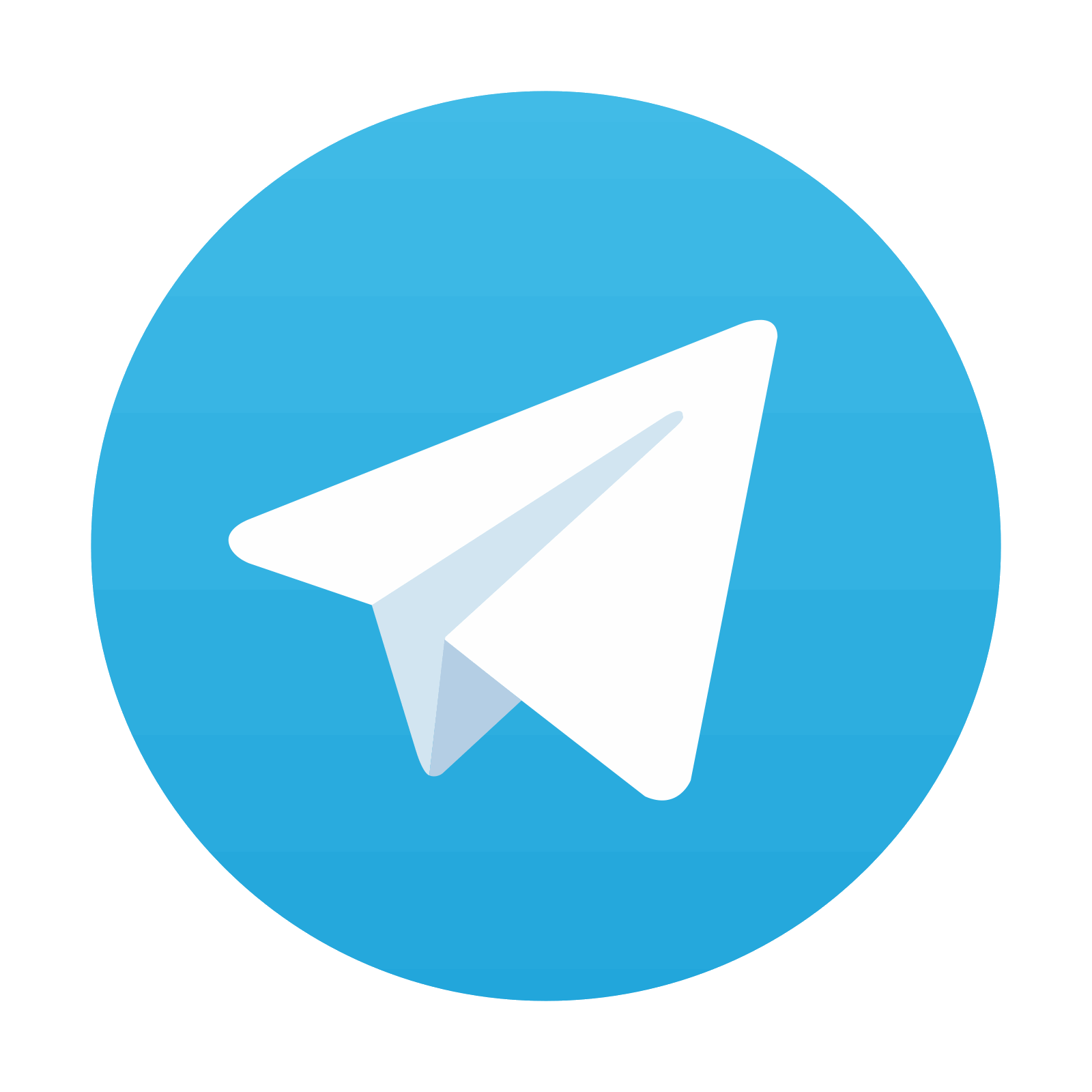
Stay updated, free articles. Join our Telegram channel
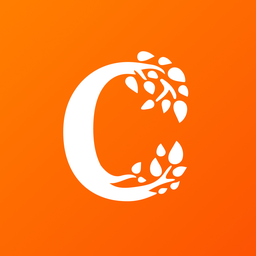
Full access? Get Clinical Tree
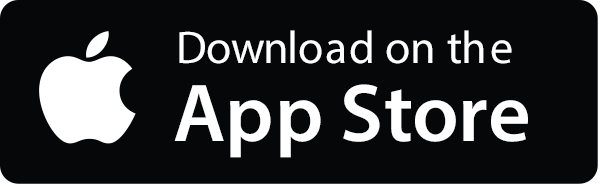
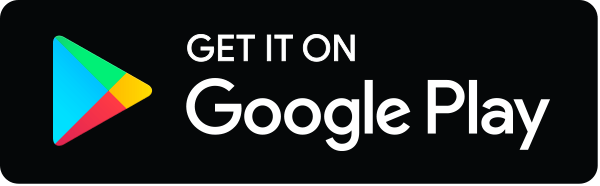