The Cerebellum
Cerebellar Diseases Have Distinctive Symptoms and Signs
The Cerebellum Has Several Functionally Distinct Regions
The Cerebellar Microcircuit Has a Distinct and Regular Organization
Neurons in the Cerebellar Cortex Are Organized into Three Layers
The Vestibulocerebellum Regulates Balance and Eye Movements
The Spinocerebellum Regulates Body and Limb Movements
The Vermis Controls Saccadic and Smooth-Pursuit Eye Movements
Spinocerebellar Regulation of Movement Follows Three Organizational Principles
The Cerebrocerebellum Is Involved in Planning Movement
Lesions of the Cerebrocerebellum Disrupt Motor Planning and Prolong Reaction Time
The Cerebrocerebellum May Have Cognitive Functions Unconnected with Motor Control
The Cerebellum Participates in Motor Learning
Climbing-Fiber Activity Produces Long-Lasting Effects on the Synaptic Efficacy of Parallel Fibers
Learning Occurs at Multiple Sites in the Cerebellar Microcircuit
THE CEREBELLUM CONSTITUTES ONLY 10% of the total volume of the brain but contains more than one-half of its neurons. The structure comprises a series of highly regular, repeating units, each of which contains the same basic microcircuit. Different regions of the cerebellum receive projections from different parts of the brain and spinal cord and project to different motor systems. Nonetheless, the similarity of the architecture and physiology in all regions of the cerebellum implies that different regions of the cerebellum perform similar computational operations on different inputs.
The symptoms of cerebellar damage in humans and experimental animals give the clear impression that the cerebellum participates in the control of movement. Thus we describe these symptoms because knowledge of them, in addition to being critical for the clinician, constrains conjecture about the exact role of the cerebellum in controlling behavior. The goal of cerebellar research is to understand how the connections and physiology of cerebellar neurons define the function of the cerebellum. Thus a major part of this chapter covers the fundamentals of cerebellar physiology and anatomy.
Finally, there is a relationship between cerebellar operation and more theoretical concepts of “internal models” in motor control (see Chapter 33). A fundamental precept of modern cerebellar research is that these internal representations of the external world are implemented in the cerebellum. The cerebellum could adjust motor performance by using its learning capabilities to alter the internal models to match any changes in the motor effectors of the external world. Thus at the conclusion of this chapter we discuss cerebellar learning and its possible relationship to internal models.
Cerebellar Diseases Have Distinctive Symptoms and Signs
Disorders of the human cerebellum result in disruptions of normal movement, described originally by Joseph Babinski in 1899 and by Gordon Holmes in the 1920s. These disruptions are in stark contrast to the paralysis caused by damage to the cerebral cortex. We cannot yet link normal cerebellar structure and function to the symptoms of cerebellar damage in humans, but the fact that movements are disrupted rather than abolished and the nature of the disruptions are important clues about cerebellar function.
Cerebellar disorders are manifested in four symptoms. The first is hypotonia, a diminished resistance to passive limb displacements. Hypotonia is also thought to be related to so-called “pendular reflexes.” The leg normally comes to rest immediately after a knee jerk produced by a tap on the patellar tendon with a reflex hammer. In patients who have cerebellar disease, however, the leg may oscillate like a pendulum as many as eight times before coming to rest.
The second symptom is astasia-abasia, an inability to stand or walk. Astasia is loss of the ability to maintain a steady limb or body posture across multiple joints. Abasia is loss of the ability to maintain upright stance against gravity. When sitting or standing, many cerebellar patients compensate by spreading their feet, an attempt to stabilize balance by increasing the base of support (see Chapter 41). They move their legs irregularly and often fall.
The third symptom is ataxia, the abnormal execution of multi-jointed voluntary movements, characterized by lack of coordination. Patients have problems initiating responses with the affected limb and controlling the size of a movement (dysmetria) and the rate and regularity of repeated movements (Figure 42–1). This last deficit, first described by Babinski, is most readily demonstrated when a patient attempts to perform rapid alternating movements, such as alternately touching the back and the palm of one hand with the palm of the other. Patients cannot sustain a regular rhythm or produce an even amount of force, a sign referred to as dysdiadochokinesia (Greek, impaired alternating movement). Holmes also noted that patients made errors in the timing of the components of complex multi-joint movements (decomposition of movement) and frequently failed to brace proximal joints against the forces generated by the movement of more distal joints.
Figure 42-1 Typical defects observed in cerebellar diseases.
A. A lesion in the left cerebellar hemisphere delays the initiation of movement. The patient is told to clench both hands at the same time on a “go” signal. The left hand is clenched later than the right, as is evident in the recordings from a pressure bulb transducer squeezed by the patient.
B. A patient moving his arm from a raised position to touch the tip of his nose exhibits inaccuracy in range and direction (dysmetria) and moves his shoulder and elbow separately (decomposition of movement). Tremor increases as the finger approaches the nose.
C. A subject was asked to alternately pronate and supinate the forearm while flexing and extending at the elbow as rapidly as possible. Position traces of the hand and forearm show the normal pattern of alternating movements and the irregular pattern (dysdiadochokinesia) typical of cerebellar disorder.
The fourth symptom of cerebellar disease is a form of tremor at the end of a movement, when the patient attempts to stop the movement by using antagonist muscles. This action (or intention) tremor is the result of a series of erroneous corrections of the movement. Once a movement is clearly headed in the wrong direction, attempts to make corrections fail repeatedly and the hand oscillates irregularly around the target in a characteristic terminal tremor. This behavior clearly suggests that the cerebellum normally is responsible for the properly timed sequence of activation in agonist and antagonist muscles and that loss of proper timing causes movements that, although initiated in the correct direction, cannot be controlled or brought to an accurate endpoint.
One conspicuous feature of cerebellar disorders is a loss of the automatic, unconscious nature of most movements, especially for motor acts made up of multiple sequential movements. One of Holmes’s patients, who had a lesion of his right cerebellar hemisphere, reported that “movements of my left arm are done subconsciously, but I have to think out each movement of the right arm. I come to a dead stop in turning and have to think before I start again.” Normally movement is controlled seamlessly by cerebellar inputs and outputs; with a malfunctioning cerebellum it seems that the cerebral cortex needs to play a more active role in programming the details of motor actions.
The Cerebellum Has Several Functionally Distinct Regions
The cerebellum occupies most of the posterior cranial fossa. It is composed of an outer mantle of gray matter (the cerebellar cortex), internal white matter, and three pairs of deep nuclei: the fastigial nucleus, the interposed nucleus (itself comprising the emboliform and globose nuclei), and the dentate nucleus (Figure 42–2A).
Figure 42-2 Gross features of the cerebellum. (Adapted, with permission, from Nieuwenhuys, Voogd, and van Huijzen 1988.)
A. Part of the right hemisphere has been cut away to reveal the underlying cerebellar peduncles.
B. The cerebellum is shown detached from the brain stem.
C. A midsagittal section through the brain stem and cerebellum shows the branching structure of the cerebellum. The cerebellar lobules are labeled with their Latin names and Larsell Roman numerals. (Reproduced, with permission, from Larsell and Jansen 1972.)
D. Functional regions of the cerebellum. (see also Figure 42-3).
The cerebellum is connected to the dorsal aspect of the brain stem by three symmetrical pairs of peduncles: the inferior cerebellar peduncle (also called the restiform body), the middle cerebellar peduncle (or brachium pontis), and the superior cerebellar peduncle (or brachium conjunctivum). Most of the output axons of the cerebellum arise from the deep nuclei and project through the superior cerebellar peduncle. The main exception is a group of Purkinje cells in the flocculonodular lobe that projects to vestibular nuclei in the brain stem.
The surface of the cerebellum is highly convoluted, with many parallel folds called folia (Latin, leaves). Two deep transverse fissures divide the cerebellum into three lobes. The primary fissure on the dorsal surface separates the anterior and posterior lobes, which together form the body of the cerebellum (Figure 42-2A). The posterolateral fissure on the ventral surface separates the body of the cerebellum from the smaller flocculo-nodular lobe (Figure 42-2B). Each lobe extends across the cerebellum from the midline to the most lateral tip.
In the orthogonal, anterior-posterior direction two longitudinal furrows divide three regions: the midline vermis (Latin, worm) and the cerebellar hemispheres, each of which is split into intermediate and lateral regions (Figure 42-2A).
The cerebellum is also divisible into three areas that have distinctive roles in different kinds of movements: the vestibulocerebellum, spinocerebellum, and cerebrocerebellum (Figure 42–3). The vestibulocerebellum consists of the flocculonodular lobe and is the most primitive part of the cerebellum, appearing first in fishes. It receives vestibular and visual inputs, projects to the vestibular nuclei in the brain stem, and participates in balance, other vestibular reflexes, and eye movements.
Figure 42-3 The three functional regions of the cerebellum have different inputs and different output targets. In the figure the cerebellum is unfolded, and arrows show the inputs and outputs of the different functional areas. The body maps in the deep nuclei are based on anatomical tracing and single-cell recordings in nonhuman primates. (D, dentate nucleus; IP, interposed nucleus; F, fastigial nucleus.) (Adapted, with permission, from Thach 1980.)
The spinocerebellum comprises the vermis and intermediate parts of the hemispheres and appears later in phylogeny. It is so named because it receives somatosensory and proprioceptive inputs from the spinal cord. The vermis receives visual, auditory, and vestibular input as well as somatic sensory input from the head and proximal parts of the body. It projects by way of the fastigial nucleus to cortical and brain stem regions that give rise to the medial descending systems controlling proximal muscles of the body and limbs. The vermis governs posture and locomotion as well as eye movements. The adjacent intermediate parts of the hemispheres also receive somatosensory input from the limbs. Neurons here project to the interposed nucleus, which provides inputs to lateral corticospinal and rubrospinal systems and controls the more distal muscles of the limbs and digits.
The cerebrocerebellum comprises the lateral parts of the hemispheres. These areas are phylogenetically most recent and are much larger in humans and apes than in monkeys and cats. Almost all of the inputs to and outputs from this region involve connections with the cerebral cortex. The output is transmitted through the dentate nucleus, which projects to motor, premotor, and pre-frontal cortices. The lateral hemispheres have many functions but seem to participate most extensively in planning and executing movement. They may also have a role in certain cognitive functions unconnected with motor planning, such as working memory. There is now some correlative evidence implicating the cerebellar hemispheres in aspects of schizophrenia (see Chapter 62) and autism (see Chapter 64).
The Cerebellar Microcircuit Has a Distinct and Regular Organization
The cellular organization of the cerebellar microcircuit is striking, and one of the premises of cerebellar research has been that the details of the microcircuit are an important clue to how the cerebellum works. Four major features of the microcircuit are described in the next four subsections.
Neurons in the Cerebellar Cortex Are Organized into Three Layers
The three layers of the cerebellar cortex possess distinct kinds of neurons and perform different operations (Figure 42–4).
Figure 42-4 The cerebellar cortex contains five types of neurons organized into three layers. A vertical section of a single cerebellar folium illustrates the general organization of the cerebellar cortex. The detail of a cerebellar glomerulus in the granular layer is also shown. A glomerulus is the synaptic complex formed by the bulbous axon terminal of a mossy fiber and the dendrites of several Golgi and granule cells. Mitochondria are present in all of the structures in the glomerulus, consistent with their high metabolic activity.
The deepest or granular layer is the input layer. It contains a vast number of granule cells, estimated at 100 billion, which appear in histological sections as small, densely packed, darkly stained nuclei. This layer also contains a few larger Golgi interneurons and, in some cerebellar regions, a smattering of other neurons such as cells of Lugaro, unipolar brush cells, and chandelier cells. The mossy fibers, one of the two principal afferent inputs to the cerebellum, terminate in this layer. The bulbous terminals of the mossy fibers excite granule cells and Golgi neurons in synaptic complexes called cerebellar glomeruli (Figure 42–4). As we will see later when discussing recurrent circuits in the cerebellum, Golgi cells inhibit granule cells.
The middle, or Purkinje cell layer, is the output layer of the cerebellar cortex. This layer consists of a single sheet of Purkinje cells bodies, which are 50 to 80 µm in diameter. The fan-like dendrites of Purkinje cells extend upward into the molecular layer where they receive inputs from the second major type of afferent fiber in the cerebellum, the climbing fibers, as well as from inhibitory and excitatory interneurons. Purkinje cell axons conduct the entire output of the cerebellar cortex, projecting to the deep nuclei in the underlying white matter or to the vestibular nuclei in the brain stem where the GABA (γ-aminobutyric acid) released by their terminals has an inhibitory action.
The outermost, or molecular layer, is an important processing layer of the cerebellar cortex. It contains the cell bodies and dendrites of two types of inhibitory interneurons, the stellate and basket cells, as well as the extensive dendrites of Purkinje cells. It also contains the axons of the granule cells, called the parallel fibers because they run parallel to the long axis of the folia (see Figure 42-4). The spatially polarized dendrites of Purkinje neurons cover extensive terrain in the anterior-posterior direction, but a very narrow territory in the medial-lateral direction. Because the parallel fibers run in the medial-lateral direction, they are oriented perpendicular to the dendritic trees of the Purkinje cells. Thus each granule cell has the potential to form a few synapses with each of a large number of Purkinje neurons, while making denser connections on a few Purkinje neurons as its axon ascends into the molecular layer.
Two Afferent Fiber Systems Encode Information Differently
The two main types of afferent fibers in the cerebellum, the mossy fibers and climbing fibers, both form excitatory synapses with cerebellar neurons but terminate in different layers of the cerebellar cortex, produce different patterns of firing in the Purkinje neurons, and thus probably mediate different functions.
Mossy fibers originate from cell bodies in the spinal cord and brain stem and carry sensory information from the periphery as well as information from the cerebral cortex. They form excitatory synapses on the dendrites of granule cells in the granular layer (Figure 42–5). Each granule cell receives inputs from just a few mossy fibers, but the architecture of the granule cell axons distributes information widely from each mossy fiber to a large number of Purkinje cells. The mossy fiber input is highly convergent; each Purkinje neuron is contacted by axons from somewhere between 200,000 and 1 million granule cells.
Climbing fibers originate in the inferior olivary nucleus and convey sensory information to the cerebellum from both the periphery and the cerebral cortex. The climbing fiber is so named because each enwraps the cell body and proximal dendrites of a Purkinje neuron like a vine on a tree, making numerous synaptic contacts (Figure 42–5). Each climbing fiber contacts 1 to 10 Purkinje neurons, but each Purkinje neuron receives synaptic input from only a single climbing fiber. The terminals of the climbing fibers are arranged topographically in the cerebellar cortex; the axons from clusters of related olivary neurons terminate in thin parasagittal strips that extend across several folia. In turn, the Purkinje neurons within one strip project to a common group of deep nuclear neurons.
Figure 42-5 Simple and complex spikes recorded intracellularly from a cerebellar Purkinje cell. Simple spikes are produced by mossy fiber input (1), whereas complex spikes are evoked by climbing fiber synapses (2). (Reproduced, with permission, from Martinez, Crill, and Kennedy 1971.)
The highly specific connectivity of the climbing fiber system contrasts markedly with the massive convergence and divergence of the mossy and parallel fibers, and suggests that the climbing fiber system is specialized for precise control of the electrical activity of Purkinje cells with related functions.
Mossy and climbing fibers have different effects on the electrical activity of Purkinje cells. Climbing fibers have an unusually powerful influence. Each action potential in a climbing fiber generates a protracted, voltage-gated Ca2+ conductance in the soma and dendrites of the postsynaptic Purkinje cell. This results in prolonged depolarization that produces a complex spike: an initial large-amplitude action potential followed by a high-frequency burst of smaller-amplitude action potentials (Figure 42–5). Whether these smaller spikes are transmitted down the Purkinje cell’s axon is not clear. In awake animals the climbing fibers spontaneously generate complex spikes at low rates, rarely more than one to three per second. When stimulated they fire single action potentials in temporal relation with specific sensory events.
The climbing-fiber system therefore seems specialized for event detection; the firing rate carries little or no information. Although climbing fibers fire only infrequently, synchronous firing in multiple climbing fibers enables them to signal important events. Synchrony seems to arise partly because neurons in the inferior olivary nucleus often are connected to one another electrotonically.
In contrast, parallel fibers produce only brief, small excitatory potentials in Purkinje neurons. These potentials spread to the initial segment of the axon where they generate simple spikes that propagate down the axon. However, inputs from many parallel fibers are needed to have a substantial effect on the frequency of simple spikes, for each postsynaptic potential is tiny. In awake animals Purkinje neurons emit a steady stream of simple spikes, with spontaneous firing rates as high as 100 per second even when an animal is sitting quietly. Purkinje neurons fire at rates as high as several hundred spikes per second during active eye, arm, and face movements, presumably because of somatosensory, vestibular, and other sensory signals that converge on granule cells through the mossy fibers. Thus the mossy-fiber system encodes the magnitude and duration of peripheral stimuli or centrally generated behaviors by controlling the firing rate of simple spikes in Purkinje cells.
Parallel Pathways Compare Excitatory and Inhibitory Signals
An important feature of the cerebellar circuit is that excitatory and inhibitory inputs are compared in both the cerebellar cortex and the deep nuclei. In the deep nuclei inhibitory inputs from Purkinje cells converge with excitatory inputs from mossy and climbing fibers (Figure 42–6).
Figure 42-6 Synaptic organization of the cerebellar microcircuit. Excitation and inhibition converge both in the cerebellar cortex and in the deep nuclei. Recurrent loops involve Golgi cells within the cerebellar cortex and the inferior olive outside the cerebellum. (Adapted, with permission, from Raymond, Lisberger, and Mauk 1996.)
The cerebellum is organized as a series of small, similar modules with close relationships among all the elements of each module. Within a given module a mossy fiber affects target neurons in the deep nuclei in two ways: directly by excitatory synapses and indirectly by pathways through the cortex and the inhibitory Purkinje cells. Thus the inhibitory output of the Purkinje cells modulates or sculpts the excitatory signals transmitted from mossy fibers to the deep nuclei. In almost all parts of the cerebellum the climbing fibers also give off collaterals that excite neurons in the deep nuclei.
In the cerebellar cortex excitatory and inhibitory inputs converge on Purkinje cells. Parallel fibers directly excite Purkinje neurons but also indirectly inhibit them through disynaptic connections from the stellate, basket, and Golgi interneurons. The short axons of stellate cells contact the nearby dendrites of Purkinje cells, whereas the long axons of basket cells run perpendicular to the parallel fibers and form synapses on the Purkinje cell bodies (Figure 42–4). The stellate cells have an inhibitory regulatory effect on the Purkinje cells that is local in that a stellate cell and the Purkinje cell it contacts are both excited by the same parallel fibers. In contrast, the basket cells create flanks of inhibition of Purkinje cells that are excited by flanking beams of parallel fibers other than the central beam that causes the lateral inhibition.
Recurrent Loops Occur at Several Levels
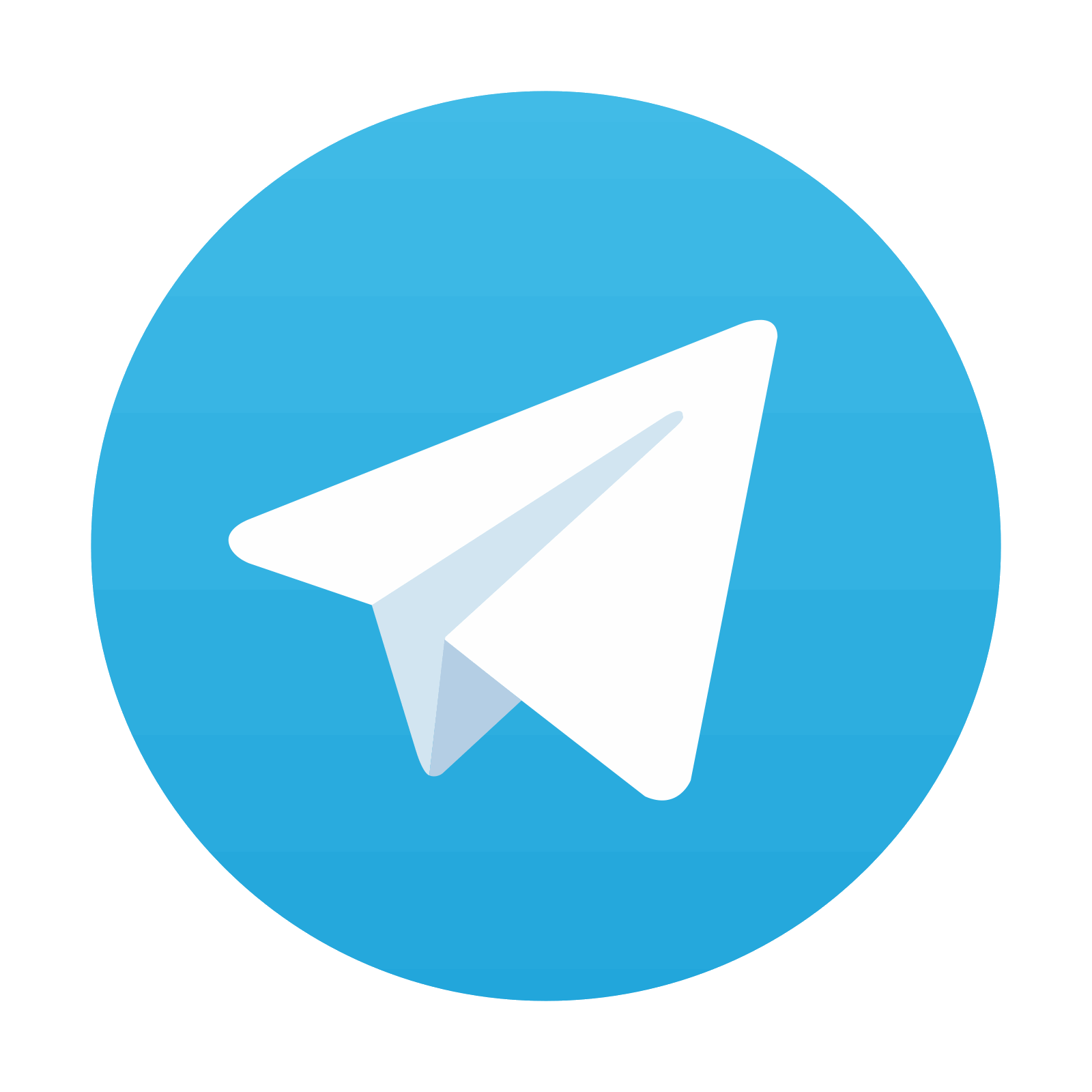
Stay updated, free articles. Join our Telegram channel
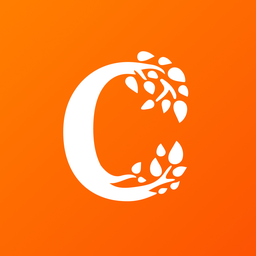
Full access? Get Clinical Tree
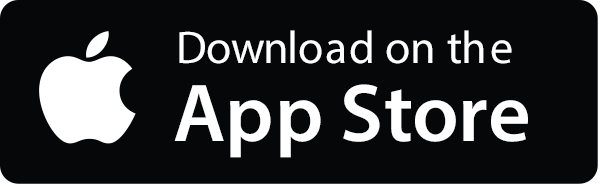
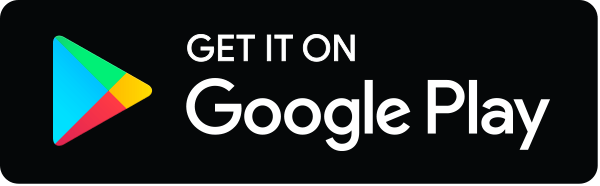