The Control of Gaze
Six Neuronal Control Systems Keep the Eyes on Target
An Active Fixation System Keeps the Fovea on a Stationary Target
The Saccadic System Points the Fovea Toward Objects of Interest
The Vergence System Aligns the Eyes to Look at Targets at Different Depths
The Eye Is Moved by the Six Extraocular Muscles
The Six Extraocular Muscles Form Three Agonist–Antagonist Pairs
The Extraocular Muscles Are Controlled by Three Cranial Nerves
The Motor Circuits for Saccades Lie in the Brain Stem
Horizontal Saccades Are Generated in the Pontine Reticular Formation
Vertical Saccades Are Generated in the Mesencephalic Reticular Formation
Brain Stem Lesions Result in Characteristic Deficits in Eye Movements
Saccades Are Controlled by the Cerebral Cortex Through the Superior Colliculus
Two Regions of Cerebral Cortex Control the Superior Colliculus
Smooth Pursuit Involves the Cerebral Cortex, Cerebellum, and Pons
Some Gaze Shifts Require Coordinated Head and Eye Movements
IN PRECEDING CHAPTERS WE LEARNED about the motor systems that control the movements of the body in space. In this and the next two chapters we consider the motor systems concerned with gaze, balance, and posture. As we explore the world around us, these motor systems act to stabilize our body, particularly our eyes. In examining these motor systems we shall be concerned with how these systems have resolved three biological challenges to knowing where we are in space: How do we visually explore our environment quickly and efficiently? How do we compensate for planned and unplanned movements of the head? How do we stay upright?
The gaze system stabilizes the image of an object on the retina when the object moves in the world or the head moves and keeps the eyes still when the image remains stationary. It has two components: the oculomotor system and the head-movement system. The oculomotor system moves the eyes in the orbits; the head-movement system moves the eye sockets.
In this chapter we describe the oculomotor system and how visual information guides eye movements. It is one of the simplest motor systems, requiring the coordination of only the 12 muscles that move the two eyes. In humans and primates the main job of the oculomotor system is to control the position of the fovea, the central, most sensitive part of the retina. The fovea is less than 1 mm in diameter and covers a tiny fraction of the visual field. When we want to examine an object, we must move its image onto the fovea.
Six Neuronal Control Systems Keep the Eyes on Target
Hermann Helmholtz and other 19th-century psycho-physicists who first studied visual perception systematically were particularly interested in eye movements. They appreciated that an analysis of eye movements was essential for understanding visual perception, but they did not realize that there is more than one kind of eye movement. In 1890 Edwin Landott discovered a second type of eye movement. When reading, the eyes do not move smoothly along a line of text but make fast, intermittent movements—saccades—each followed by a short pause (see Chapter 29).
By 1902 Raymond Dodge was able to outline five distinct types of eye movement that direct the fovea to a visual target and keep it there. All of these eye movements share an effector pathway originating in the three bilateral groups of oculomotor neurons in the brain stem.
• Saccadic eye movements shift the fovea rapidly to a new visual target.
• Smooth-pursuit movements keep the image of a moving target on the fovea.
• Vergence movements move the eyes in opposite directions so that the image is positioned on both foveae.
• Vestibulo-ocular reflexes hold images still on the retina during brief head movements.
• Optokinetic movements hold images stationary during sustained head rotation or translation.
A sixth system, the fixation system, holds the eye stationary during intent gaze when the head is not moving. This requires active suppression of eye movement. The optokinetic and vestibular systems are discussed in Chapter 40; we consider the remaining four systems here.
An Active Fixation System Keeps the Fovea on a Stationary Target
Vision is most accurate when the eyes are still. The gaze system actively prevents the eyes from moving when we examine an object of interest. It is not as active in suppressing movement when we are doing something that does not require vision, such as mental arithmetic. Patients with disorders of the fixation system—for example, some individuals with congenital nystagmus—have poor vision not because their visual acuity is deficient but because they cannot hold their eyes still enough for the visual system to work correctly.
The Saccadic System Points the Fovea Toward Objects of Interest
Our eyes explore the world in a series of very quick saccades that move the fovea from one fixation point to another (Figure 39–1). Saccades allow us to scan the environment quickly and to read. They are highly stereotyped; they have a standard waveform with a single smooth increase and decrease of eye velocity. They are also extremely fast, occurring within a fraction of a second at angular speeds up to 900 degrees per second (Figure 39-2A). The velocity of a saccade is determined by only the distance of the target from the fovea. We can change the amplitude and direction of saccades voluntarily but not their speed.
Figure 39–1 Eye movements track the outline of an object of attention. An observer looks at a picture of a woman for 1 minute. The resulting eye positions are then superimposed on the picture. As shown here, the observer concentrated on certain features of the face, lingering over the woman’s eyes and mouth (fixations) and spending less time over intermediate positions. The rapid movements between fixation points are saccades. (Reproduced, with permission, from Yarbus 1967.)
Figure 39–2 Saccadic and smooth-pursuit eye movements. Eye position, target position, and eye velocity are plotted against time.
A. The human saccade. At the beginning of the plot the eye is on the target (the traces representing eye and target positions are superimposed). Suddenly the target jumps to the right, and within 200 ms the eye moves to bring the target back to the fovea. Note the smooth, symmetric velocity profile. Because eye movements are rotations of the eye in the orbit, they are described by the angle of rotation. Similarly, objects in the visual field are described by the angle of arc they subtend at the eye. Viewed at arm’s length, a thumb subtends an angle of approximately 1 degree. A saccade from one edge of the thumb to the other therefore traverses 1 degree of arc.
B. Human smooth pursuit. In this example the subject is asked to make a saccade to a target that jumps away from the center of gaze and then slowly moves back to center. The first movement seen in the position and velocity traces is a smooth-pursuit movement in the same direction as the target movement. The eye briefly moves away from the target before a saccade is initiated because the latency of the pursuit system is shorter than that of the saccade system. The smooth-pursuit system is activated by the target moving back toward the center of gaze, the saccade adjusts the eye’s position to catch the target, and thereafter smooth pursuit keeps the eye on the target. The recording of saccade velocity is clipped so that the movement can be shown on the scale of the pursuit movement, an order of magnitude slower than the saccade.
Ordinarily there is no time for visual feedback to modify the course of a saccade; corrections to the direction of movement are made in successive saccades. Only fatigue, drugs, or pathological states can slow saccades. Accurate saccades can be made not only to visual targets but also to sounds, tactile stimuli, memories of locations in space, and even verbal commands (“look left”).
The Smooth-Pursuit System Keeps Moving Targets on the Fovea
The smooth-pursuit system holds the image of a moving target on the fovea by calculating how fast the target is moving and moving the eyes at the same speed. Smooth-pursuit movements have a maximum angular velocity of approximately 100 degrees per second, much slower than saccades. Drugs, fatigue, alcohol, and even distraction degrade the quality of these movements.
Smooth pursuit and saccades have very different central control systems. This is best seen when a target jumps away from the center of gaze and then slowly moves back toward it. A smooth-pursuit movement is initiated first because the smooth-pursuit system has a shorter latency and responds to target motion on the peripheral retina as well as on the fovea. As the target moves back toward the center of gaze, the eye briefly moves away from the target before the saccade is initiated (Figure 39-2B). The subsequent saccade then brings the eye to the target.
The Vergence System Aligns the Eyes to Look at Targets at Different Depths
The smooth-pursuit and saccade systems produce conjugate eye movements: Both eyes move in the same direction and at the same speed. In contrast, the vergence system produces disconjugate movements of the eyes. When we look at an object that is close to us, our eyes rotate toward each other, or converge; when we look at an object that is farther away, they rotate away from each other, or diverge (Figure 39–3). These disconjugate movements ensure that the image of the object falls on the foveae of both retinas. Whereas the visual system uses slight differences in left and right retinal positions, or retinal disparity, to create a sense of depth, the vergence system drives disconjugate movements to eliminate retinal disparity at the fovea.
Figure 39–3 Vergence movements. When the eyes focus on a distant mountain, images of the mountain lie on the foveae, whereas those of the nearer tree occupy different retinal positions relative to the two foveae, yielding the percept of a double image. When the viewer looks instead at the tree (below), the vergence system must rotate each eye inward. Now the tree’s image occupies similar positions on the foveae of both retinas and is seen as one object, but the mountain’s images occupy different locations on the retinas and appear double. (Reproduced, with permission, from F. A. Miles.)
Vergence is a function of the horizontal rectus muscles only. Near-field viewing is accomplished by simultaneously increasing the tone of the medial recti muscles and decreasing the tone of the lateral recti muscles. Distance viewing is accomplished by reducing medial-rectus tone and increasing lateral-rectus tone. Accommodation and vergence are controlled by midbrain neurons in the region of the oculomotor nucleus. Neurons in this region discharge during vergence, accommodation, or both.
At any given time the entire visual field is not in focus on the retina. When we look at something close by, distant objects are blurred. When we look at something far away, near objects are blurred. When we wish to focus on an object in a closer plane in the visual field, the oculomotor system contracts the ciliary muscle, thereby changing the radius of curvature of the lens. This process is called accommodation. In older individuals accommodation declines owing to increased rigidity of the lens; reading glasses are then needed to focus images at short distances.
Accommodation and vergence are linked. Accommodation is elicited by the blurring of an image, and whenever accommodation occurs the eyes also converge. Conversely, retinal disparity induces vergence, and whenever the eyes converge, accommodation also takes place. At the same time, the pupils transiently constrict to increase the depth of field of the focus. The linked phenomena of accommodation, vergence, and pupillary constriction comprise the near response.
The neural signal sent to each eye muscle has two components, one related to eye position and the other to eye velocity. Velocity and position signals are generated by different neural systems that converge on the motor neuron. In addition, horizontal and vertical eye movements are specified independently; vertical movements are generated in the mesencephalic reticular formation and horizontal movements in the pontine reticular formation.
Inhibitory neurons suppress unwanted eye movements. Omnipause neurons in the pontine reticular formation prevent excitatory neurons in the brain stem from stimulating the motor neurons. Fixation neurons in the rostral superior colliculus inhibit movement-related neurons in the colliculus while exciting omnipause neurons in the pons. Inhibitory neurons in the substantia nigra inhibit these same movement-related neurons from firing except during saccades.
The Eye Is Moved by the Six Extraocular Muscles
Eye Movements Rotate the Eye in the Orbit
To a good approximation, the eye is a sphere that sits in a socket, the orbit. Eye movements are simply rotations of the eye in the orbit. The eye’s orientation can be defined by three axes of rotation—horizontal, vertical, and torsional—that intersect at the center of the eyeball, and eye movements are described as rotations around these axes. Horizontal and vertical eye movements change the line of sight by redirecting the fovea; torsional eye movements rotate the eye around the line of sight but do not change gaze.
Horizontal rotation of the eye away from the nose is called abduction and rotation toward the nose is adduction. Vertical movements are referred to as elevation (upward rotation) and depression (downward rotation). Finally, torsional movements include intorsion (rotation of the top of the cornea toward the nose) and extorsion (rotation away from the nose).
Except for vergence, most eye movements are conjugate. For example, during gaze to the right the right eye abducts and the left eye adducts. Similarly, if the right eye extorts, the left eye intorts.
The Six Extraocular Muscles Form Three Agonist–Antagonist Pairs
Each eye is rotated by six extraocular muscles arranged in three agonist–antagonist pairs (Figure 39–4). The four rectus muscles (lateral, medial, superior, and inferior) share a common origin, the annulus of Zinn, at the apex of the orbit. They insert on the surface of the eye, or sclera, anterior to the eye’s equator. The origin of the inferior oblique muscle is on the medial wall of the orbit; the superior oblique muscle’s tendon passes through the trochlea, or pulley, before inserting on the globe, so that its effective origin is also on the medial wall of the orbit. The oblique muscles insert on the posterior globe.
Figure 39–4 The origins and insertions of the extraocular muscles.
A. Lateral view of the left eye with the orbital wall cut away. Each rectus muscle inserts in front of the equator of the globe so that contraction rotates the cornea toward the muscle. Conversely, the oblique muscles insert behind the equator and contraction rotates the cornea away from the insertion. The superior oblique muscle passes through a bony pulley, the trochlea, before it inserts on the globe. The levator muscle of the upper eyelid raises the lid.
B. Superior view of the left eye with the roof of the orbit and the levator muscle cut away. The superior rectus passes over the superior oblique and inserts in front of it on the globe.
Each muscle has a dual insertion. The part of the muscle farthest from the eye inserts on a soft-tissue pulley through which the rest of the muscle passes on its way to the eye. When the extraocular muscles contract, they not only rotate the eye but also change their pulling directions.
The actions of the extraocular muscles are determined by their geometry and by the position of the eye in the orbit. The medial and lateral recti rotate the eye horizontally; the medial rectus adducts, whereas the lateral rectus abducts. The superior and inferior recti and the obliques rotate the eye both vertically and torsionally. The superior rectus and inferior oblique elevate the eye, and the inferior rectus and superior oblique depress it. The superior rectus and superior oblique intort the eye, whereas the inferior rectus and inferior oblique extort it.
The relative amounts of vertical and torsional rotation produced by the superior and inferior recti and the obliques depend on eye position. The superior and inferior recti exert their maximal vertical action when the eye is abducted, that is, when the line of sight is parallel to the muscles’ pulling directions. Conversely, the oblique muscles exert their maximal vertical action when the eye is adducted (Figure 39–5).
Figure 39–5 Each superior rectus and oblique muscle has both torsional and elevational actions. How much elevation and torsion each muscle provides depends on the position of the eye. (Adapted, with permission, from von Noorden 1980.)
A. When eye position is in the primary visual axis (the y axis in the diagram) or lateral to it (abduction), elevation is provided by the superior rectus and all of the intorsion is from the superior oblique muscle. When the eye is positioned completely medial to the visual axis (adduction), intorsion comes predominantly from the superior rectus and most of the elevation comes from the inferior oblique.
B. When the eye is positioned 16 degrees or more laterally from the primary visual axis, the superior oblique intorts the eye and depression comes from the inferior rectus. When it is completely medial to the visual axis, the superior oblique rotates the eye vertically downward and all of the intorsion comes from the superior rectus.
Movements of the Two Eyes Are Coordinated
Humans and other animals with eyes in front have binocular vision. This facilitates stereopsis, the ability to perceive a visual scene in three dimensions, as well as depth perception. At the same time, binocular vision requires precise coordination of the movements of the two eyes so that both foveae are always directed at the target of interest. For most eye movements both eyes must move by the same amount and in the same direction. This is accomplished, in large part, through the pairing of eye muscles in the two eyes.
Just as each eye muscle is paired with its antagonist in the same orbit (eg, the medial and lateral recti), it is also paired with the muscle that moves the opposite eye in the same direction. For example, coupling of the left lateral rectus and right medial rectus moves both eyes to the left during a leftward saccade. The orientations of the vertical muscles are such that each pair consists of one rectus muscle and one oblique muscle. For example, the left superior rectus and the right inferior oblique both move the eyes upward in left gaze. The binocular muscle pairs are listed in Table 39-1.
Figure 39-6 Vertical Muscle Action in Adduction and Abduction
The Extraocular Muscles Are Controlled by Three Cranial Nerves
The extraocular muscles are innervated by groups of motor neurons whose cell bodies are clustered in three nuclei in the brain stem (Figure 39–6). The lateral rectus is innervated by the abducens nerve (cranial nerve VI), whose nucleus lies in the pons in the floor of the fourth ventricle. The superior oblique muscle is innervated by the trochlear nerve (cranial nerve IV), whose nucleus is located in the midbrain at the level of the inferior colliculus. (The trochlear nerve gets its name from the trochlea, the bony pulley through which the superior oblique muscle travels.)
Figure 39–6 The ocular motor nuclei in the brain stem. The nuclei are shown in a parasagittal section through the thalamus, pons, midbrain, and cerebellum of a rhesus monkey. The oculomotor nucleus (cranial nerve III) lies in the midbrain at the level of the mesencephalic reticular formation. The trochlear nucleus (nerve IV) is slightly caudal, and the abducens nucleus (nerve VI) lies in the pons at the level of the paramedian pontine reticular formation, adjacent to the fasciculus of the facial nerve (VII). Compare Figure 45-5). (iC, interstitial nucleus of Cajal; iMLF, interstitial nucleus of the medial longitudinal fasciculus; nD, nucleus of Darkshevich; VN, vestibular nuclei.) (Adapted, with permission, from Henn et al. 1984.)
All the other extraocular muscles—the medial, inferior, and superior recti and the inferior oblique—are innervated by the oculomotor nerve (cranial nerve III), whose nucleus lies in the midbrain at the level of the superior colliculus. The oculomotor nerve also contains fibers that innervate the levator muscle of the upper eyelid. Cell bodies of axons innervating both eyelids are located in the central caudal nucleus, a single midline structure within the oculomotor complex. Finally, traveling with the oculomotor nerve are parasympathetic fibers that innervate the iris sphincter muscle, the constrictor of the pupil, and the ciliary muscles that adjust the curvature of the lens to focus the eye during accommodation.
The pupil and eyelid also have sympathetic innervation, which originates in the intermediolateral cell column of the ipsilateral upper thoracic spinal cord. Fibers of these neurons synapse on cells in the superior cervical ganglion in the upper neck. Axons of these postganglionic cells travel along the carotid artery to the carotid sinus and then into the orbit. Sympathetic pupillary fibers innervate the iris dilator muscle, causing the pupil to dilate and thus providing the pupillary component of the so-called “fight or flight” response. Sympathetic fibers also innervate Müller’s muscle, a secondary elevator of the upper eyelid. The sympathetic control of pupillary dilatation and lid elevation is responsible for the “wide-eyed” look of excitement and sympathetic overload.
The best way to understand the actions of the extraocular muscles is to consider the eye movements that remain after a lesion of a specific nerve (Box 39–1).
Extraocular Motor Neurons Encode Eye Position and Velocity
We can illustrate how the gaze system generates eye movements by considering the activity of an oculomotor neuron during a saccade. To move the eye quickly to a new position in the orbit and keep it there, two passive forces must be overcome: the elastic force of the orbit, which tends to restore the eye to a central position in the orbit, and a velocity-dependent viscous force that opposes rapid movement. Thus the motor signal must include information about tonic position, which opposes the elastic force, and velocity, which overcomes orbital viscosity and moves the eye quickly to a new position.
Information about the position and velocity of the eye is conveyed by the discharge frequency of an oculomotor neuron (Figure 39–8). The firing rate of the neuron rises rapidly as the eye’s velocity increases from 0 degrees to 900 degrees per second; this is called the saccadic pulse. The frequency of this pulse determines the speed of the saccade, whereas the duration of the pulse controls the duration of the saccade. The difference in the firing rates before and after the saccade is called the saccadic step. As described below, the pulse and step are generated by different brain stem structures.
Figure 39–8 Oculomotor neurons signal eye position and velocity.
A. The record is from an abducens neuron of a monkey. When the eye is positioned in the medial side of the orbit the cell is silent (position θ0). As the monkey makes a lateral saccade there is a burst of firing (D1), but in the new position θ1) the eye is still too far medial for the cell to discharge continually. During the next saccade there is a burst (D2), and at the new position θ2) there is a tonic position-related discharge. Before and during the next saccade (D3) there is again a pulse of activity and a higher tonic discharge when the eye is at the new position θ4). When the eye makes a medial movement there is a period of silence during the saccade (D4) even though the eye ends up at a position associated with a tonic discharge. (Adapted, with permission, from A. Fuchs 1970.)
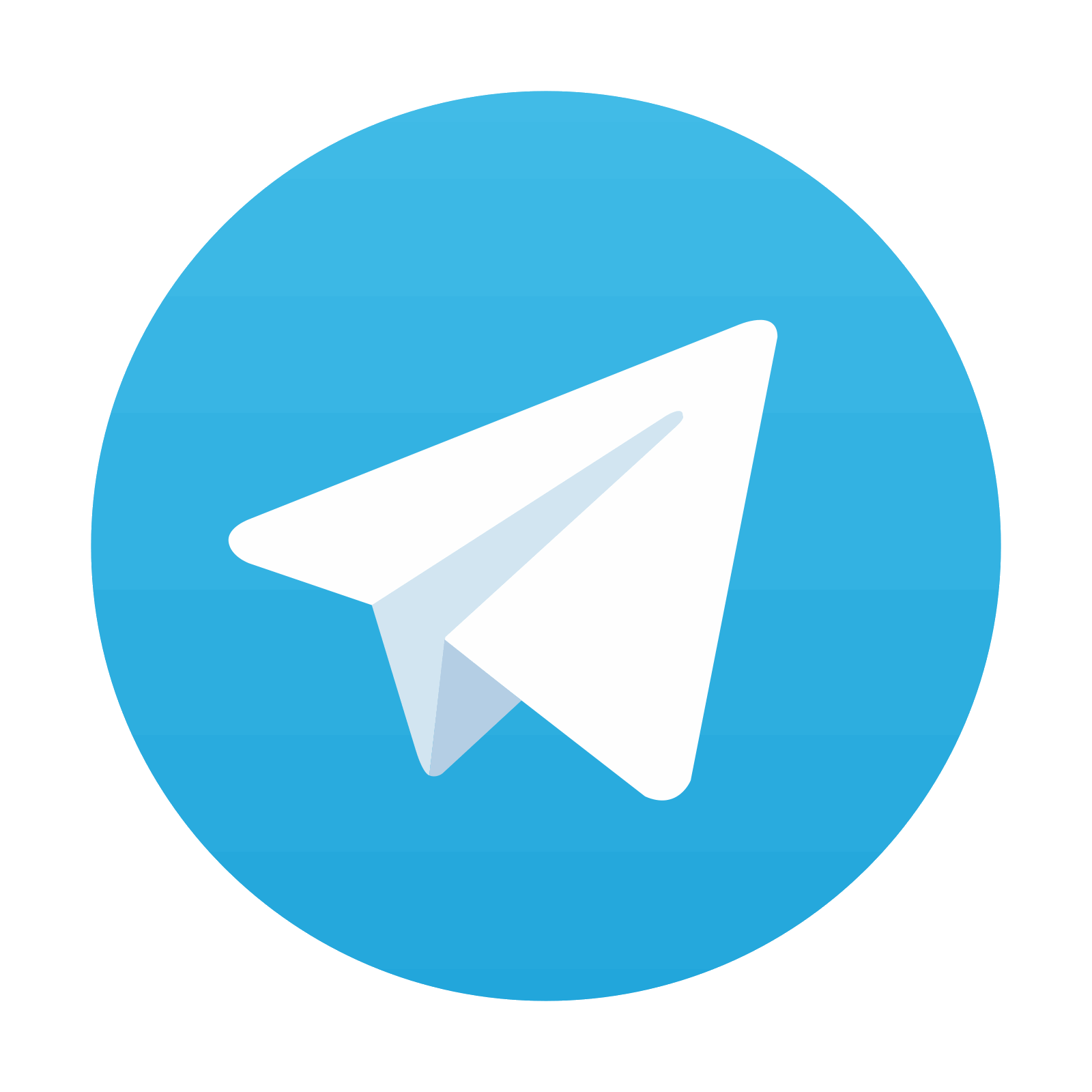
Stay updated, free articles. Join our Telegram channel
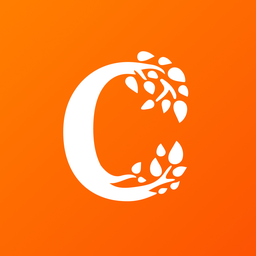
Full access? Get Clinical Tree
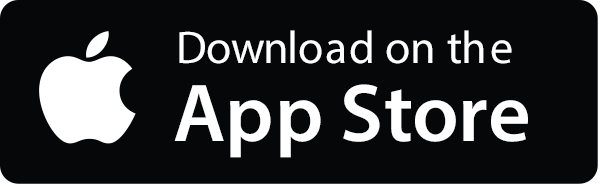
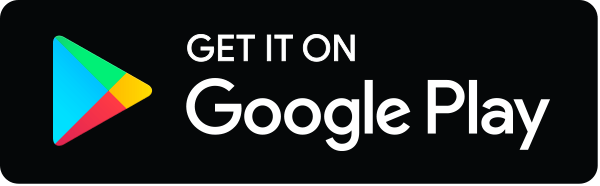