The Growth and Guidance of Axons
Differences in the Molecular Properties of Axons and Dendrites Emerge Early in Development
Neuronal Polarity Is Established Through Rearrangements of the Cytoskeleton
The Growth Cone Is a Sensory Transducer and a Motor Structure
Molecular Cues Guide Axons to Their Targets
The Growth of Retinal Ganglion Axons Is Oriented in a Series of Discrete Steps
Ephrins Provide Gradients of Inhibitory Signals in the Brain
Axons from Some Spinal Neurons Cross the Midline
Netrins Direct Developing Commissural Axons Across the Midline
Chemoattractant and Chemorepellent Factors Pattern the Midline
IN THE TWO PRECEDING CHAPTERS we saw how neurons are generated in appropriate numbers, at correct times, and in the right places. These early developmental steps set the stage for later events that direct neurons to form functional connections with target cells. To form connections neurons have to extend long processes—axons and dendrites—which permit connectivity with postsynaptic cells and synaptic input from other neurons.
The growing axon may have to travel a long distance—up to several meters in a giraffe—and ignore many inappropriate neuronal partners before terminating in just the right region and recognizing its correct synaptic targets. In this chapter we examine how neurons elaborate axons and dendrites, and how axons are guided to their targets. In subsequent chapters we consider how neurons form synapses and how patterns of connections are shaped by activity.
We begin this chapter by discussing how certain neuronal processes become axons and others dendrites. We then consider the challenges that face an axon as it projects along tortuous pathways to its target. Finally, we illustrate general features of axonal guidance by describing the development of two well-studied axonal pathways: one that conveys visual information from the retina to the brain and another that conveys cutaneous sensory information from the spinal cord to the brain.
Differences in the Molecular Properties of Axons and Dendrites Emerge Early in Development
The processes of neurons vary enormously in their length, thickness, branching pattern, and molecular architecture. Nonetheless, most neuronal processes fit two functional categories: axons and dendrites. More than a century ago Santiago Ramón y Cajal hypothesized that this distinction underlies the ability of neurons to transmit information in a particular direction, an idea he formalized as the law of dynamic polarization. Cajal wrote that “the transmission of the nerve impulse is always from the dendritic branches and the cell body to the axon.” In the decades before electrophysiological methods were up to the task, this law provided a means of analyzing neural circuits histologically. Although exceptions have been found, Ramón y Cajal’s law remains a basic principle that relates structure and function in the nervous system and highlights the importance of knowing how neurons acquire their polarized form.
Neuronal Polarity Is Established Through Rearrangements of the Cytoskeleton
Much of our knowledge about neuronal polarization comes from studies of neurons taken from the rodent brain and grown in tissue culture. Hippocampal neurons grown in isolation develop processes reminiscent of those seen in vivo: a single, long, cylindrical axon and several shorter, tapered dendrites (Figure 54-1A). Axons and dendrites soon acquire molecular distinctions, as cytoskeletal and synaptic proteins are targeted to these components. For example, a particular form of the Tau protein is localized in axons and the MAP2 protein in dendrites (Figure 54-1B)
Figure 54-1 The differentiation of axons and dendrites marks the emergence of neuronal polarity.
A. Four stages in the polarization of a hippocampal neuron grown in tissue culture. (Adapted, with permission, from Kaech and Banker 2006.)
B. Hippocampal neurons grown in culture possess multiple short, thick dendrites that are enriched in the microtubule-associated protein MAP2. They also possess a single long axon that is marked by a dephosphorylated form of the microtubule-associated protein tau (left). A cultured neuron isolated from a mutant mouse lacks expression of a Par family gene (SAD kinase). The neuron generates neurites that co-express Tau and MAP2, markers of axons and dendrites respectively. The length and diameter of these neurites are intermediate in size between those of axons and dendrites (right). (Reproduced, with permission, from Kishi et al. 2005.)
Cultured neurons are especially useful for developmental studies because they initially show no obvious sign of polarization and acquire their specialized features gradually in a stereotyped sequence of cellular steps. This sequence begins with extension of several short processes, each equivalent to the others. Soon thereafter, one process is established as an axon and the remaining processes acquire dendritic features (Figure 54-1A).
How does this occur? Cytoskeletal proteins that maintain elongated processes and drive growth are central to this process. If the actin filaments in an early neurite are destabilized, the ensuing cytoskeletal rearrangements commit the neurite to becoming the axon, while the remaining neurites become dendrites. If the nascent axon is removed, one of the remaining neurites quickly assumes an axonal character. This sequence suggests that axonal specification is a key event in neuronal polarization and that signals from newly formed axons both suppress the generation of additional axons and promote dendrite formation.
The nature of the axonally derived signal that represses other axons is not known. But some insight into signals that control cytoskeletal arrangements has come from the study of a group of proteins encoded by the Par complex genes. As first shown in the nematode worm Caenorhabditis elegans, Par proteins are involved in diverse aspects of cytoskeletal reorganization, including the polarization of neuronal processes. Mammalian forebrain neurons lacking Par3, Par4, Par6, or relatives of Par1 grow multiple processes that are intermediate in length between axons and dendrites and bear markers of both processes (Figure 54-1B).
Although neurons grown in culture are similar to those in the brain, they are deprived of key extrinsic cues and signals. Cultured neurons become randomly arranged with respect to each other, whereas in many regions of the developing brain neurons line up in rows, with their dendrites pointing in the same direction (Figure 54-2A). This difference in vivo and in vitro implies that extrinsic signals regulate the polarization machinery. In the developing brain the local release of semaphorins, and other axonal guidance factors that we discuss later in the chapter, may help to orient dendrites (Figure 54-2C). The job of the Par protein complex is to link these extracellular signals to the cellular machinery that rearranges the cytoskeleton, a process achieved in part through the regulation of proteins that modify actin or tubulin function. In fact both the Tau protein in axons and the MAP2 protein in dendrites associate with and affect microtubules.
Figure 54-2 Extracellular factors determine whether neuronal processes become axons or dendrites.
A. Cortical pyramidal neurons in vivo display a common axonal and dendritic orientation. (Image reproduced, with permission, from Josh Sanes.)
B. Neurons growing on laminin acquire polarity. When a cortical neuron extends a process from a less attractive substrate onto laminin, the process grows faster and usually becomes an axon. (Image reproduced, with permission, from Paul Letourneau.)
C. In the developing neocortex semaphorin-3A (Sema 3A) is secreted by cells near the pial surface. Semaphorin-3A is an attractant for growing dendrites, helping to establish neuronal polarity and orientation. The parallel orientation of cortical pyramidal neurons is disrupted in mutant mice lacking functional semaphorin-3A. (Reproduced, with permission, from Polleux, Morrow, and Ghosh 2000.)
If local signals are needed to polarize neurons in the brain, how is polarity established in the uniform environment of a tissue culture? One possible explanation is that minor variations in the intensity of signaling within a neuron, or in signals from its immediate environment, will activate Par proteins in one small domain of the neuron, triggering the nearest process to become an axon. If, by happenstance, one process grows slightly faster than its neighbors, its chances of becoming an axon increase markedly (Figure 54-2B). Presumably, this proto-axonal process emits signals that decrease the chance of other processes following suit, forcing them to become dendrites.
Dendrites Are Patterned by Intrinsic and Extrinsic Factors
Although processes destined to become axons and dendrites initially are indistinguishable, they soon acquire distinct features. Nascent dendrites grow at acute angles and their branches are generally more numerous and closer to the cell body than those of axons. In addition, small protrusions called spines extend from the distal branches of dendrites. Finally, some dendritic branches are retracted or “pruned” to give the arbor its final and definitive shape (Figure 54-3).
Figure 54-3 Dendritic branching develops in a series of steps. The outgrowth of dendrites involves the formation of elaborate branches from which spines develop. Certain branches and spines are later pruned to achieve the mature pattern of dendrite arborization. (Image of spines at right reproduced, with permission, from Stefan W. Hell.)
Although the core features of dendrite formation are common to many neurons, there is a striking variation in dendrite number, shape, and branching pattern among neuronal types. Indeed, the shape and form of its dendritic arbor is one of the main ways in which neurons can be classified. Cerebellar Purkinje cells can be distinguished from granule cells, spinal motor neurons, and hippocampal pyramidal neurons simply by looking at the pattern of their dendrites.
How is dendritic pattern established? Neurons must have intrinsic information about their shape because the patterns in tissue culture are strikingly reminiscent of those in vivo (Figure 54-4). The transcriptional programs that specify neuronal subtype (see Chapter 52) presumably also encode information about neuronal shape. A second mechanism for establishing the pattern of dendritic arbors is the recognition of one dendrite by others of the same cell. In the Drosophila nervous system repellent signaling between dendrites of the same neuron, mediated by the different isoforms of a recognition protein known as DS-CAM, helps to ensure that dendrites expand over a broad territory rather than bunching together.
Figure 54-4 The morphologies of neurons are preserved in dissociated cell culture. Cerebellar Purkinje neurons and hippocampal pyramidal neurons have distinctive patterns of dendritic branching. These basic patterns are recapitulated when these two classes of neurons are isolated and grown in dissociated cell culture. (The image on the upper left is from David L. Becker; upper right from Yoshio Hirabayashi; lower left by Grazyna Gorney, reproduced, with permission, from Terry E. Robinson; the image in the lower right from Kelsey Martin. All images reproduced with permission.)
The dendrites of neighboring neurons also provide cues. In many cases the dendrites of a particular class of neuron cover a surface with minimal overlap, a spacing pattern called tiling. The tiling of dendrites appears to result from specific inhibitory interactions among the dendrites of a particular class of neuron. Tiling allows each class of neuron to receive information from the entire surface or area it innervates. Tiling of a region by the dendrites of one class of neuron also avoids the confusion that could arise if the dendrites of many different neurons occupied the same area.
Thus interactions between dendrites establish an overall arborization pattern through a mixture of intrinsic and extracellular mechanisms. Once a cell process is committed to an axonal or dendritic character, it becomes sensitive to a variety of intrinsic and extrinsic signals that determine its trajectory. For dendrites these patterning signals determine neuronal morphology. For axons, which we consider next, the signals guide the axons to their targets.
The Growth Cone Is a Sensory Transducer and a Motor Structure
Once an axon forms it begins to grow toward its synaptic target. The key neuronal element responsible for axonal growth is a specialized structure at the tip of the axon called the growth cone. Both axons and dendrites use growth cones for elongation, but those linked to axons have been studied more intensively.
Ramón y Cajal discovered the growth cone and had the key insight that it was responsible for axonal pathfinding. With static images alone for inspiration (Figure 54-5A), he envisioned the growth cone to be “endowed with exquisite chemical sensitivity, rapid ameboid movements and a certain motive force, thanks to which it is able to proceed forward and overcome obstacles met in its way … until it reaches its destination.”
Figure 54-5 Neuronal growth cones.
A. Drawings of growth cones by Santiago Ramón y Cajal, who discovered these cellular structures and inferred their function.
B. The diverse morphologies of growth cones are visualized in dye-labeled retinal ganglion neurons in the mouse. Note the similarities with Cajal’s drawings. (Reproduced, with permission, from Carol Mason and Pierre Godemont.)
C. The three main domains of the growth cone—filopodia, lamellipodia, and a central core—shown by whole-mount electron microscopy. (Reproduced, with permission, from Bridgman and Dailey 1989.)
D. The growth cone of a neuron from Aplysia in which actin and tubulin have been visualized. Actin (purple) is concentrated in lamellipodia and filopodia, whereas tubulin and microtubules (aquamarine) are concentrated in the central core. (Image reproduced, with permission, from Paul Forscher and Dylan Burnette.)
Many studies over the past century have confirmed Ramón y Cajal’s intuition. We now know that the growth cone is both a sensory structure that receives directional cues from the environment and a motor structure whose activity drives axon elongation. Ramón y Cajal also pondered “what mysterious forces precede the appearance of these processes … promote their growth and ramification … and finally establish those protoplasmic kisses … which seem to constitute the final ecstasy of an epic love story.” In more modern and prosaic terms we now know that the growth cone guides the axon by transducing positive and negative cues into signals that regulate the cytoskeleton, thereby determining the course and rate of axonal outgrowth.
Growth cones have three main compartments. Their central core is rich in microtubules, mitochondria, and other organelles. Long slender extensions called filopodia project from the body of the growth cone. Between the filopodia lie lamellipodia, which are also motile and give the growth cone its characteristic ruffled appearance (Figure 54-5C).
Growth cones sense environmental signals through their filopodia: rod-like, actin-rich, membrane-limited structures that are highly motile. Their surface membranes bear receptors for the molecules that serve as directional cues for the axon. Their length—tens of micrometers in some cases—permits the filopodia to sample environments far in advance of the central core of the growth core. Their rapid movements permit them to compile a detailed inventory of the environment, and their flexibility permits them to navigate around cells and other obstacles.
When filopodia encounter signals in the environment, the growth cone is stimulated to advance, retract, or turn. Several motors power these orienting behaviors. One source of power is the movement of actin along myosin, an interaction similar to the one that powers the contraction of skeletal muscle fibers, although the actin and myosin of neurons are different from those in muscle. The assembly of actin monomers into polymeric filaments also contributes a propulsive force for filopodial extension. Acting in parallel, actin filaments constantly depolymerize at the base of filopodia. Depolymerization slows during periods of growth cone advance, leading to greater net forward motion. The movement of membranes along the substrate provides yet another source of forward motion.
The contribution of each type of molecular motor to the advance of the growth cone is likely to vary from one situation to another. With all of these motors the final step involves the flow of microtubules from the central core of the growth cone into the newly extended tip, thus moving the growth cone ahead and leaving in its wake a new segment of axon. New lamellipodia and filopodia form in the advancing growth cone and the cycle repeats (Figure 54-6).
Figure 54-6 The growth cone advances under the control of cellular motors. (Modified, with permission, from Heidmann 1996.)
A. A filopodium contacts an adhesive cue and contracts, thus pulling the growth cone forward (1). Actin filaments assemble at the leading edge of a filopodium, disassemble at the trailing edge, and interact with myosin along the way (2). Actin polymerization pushes the filopodium forward (3). Force generated by the retrograde flow of actin pushes the filopodium forward. Exocytosis adds membrane to the leading edge of the filopodium and supplies new adhesion receptors to maintain traction. Membrane is recovered at the back of the filopodium. The actin polymer is linked to adhesion molecules on the plasma membrane.
B. The combined action of these motors creates an actin-depleted space that is filled by the advance of microtubules from the central core.
C. Individual microtubules condense to form a thick bundle, and the cytoplasm collapses around them to create a new segment of axonal shaft.
Accurate pathfinding can occur only if the growth cone’s motor action is linked to its sensory function. It is crucial therefore that the recognition proteins on the filopodia are signal-inducing receptors and not merely binding moieties that mediate adhesion. The binding of a ligand to its receptor affects growth in diverse ways. It stimulates the formation, accumulation, and even the breakdown of soluble intracellular molecules that function as second messengers. These second messengers affect the organization of the cytoskeleton, and in this way regulate the direction and rate of movement of the growth cone.
One important second messenger is calcium. The calcium concentration in growth cones is regulated by the activation of receptors on filopodia and this affects the organization of the cytoskeleton, which in turn modulates motility. Growth cone motility is optimal within a narrow range of calcium concentrations, called a set point. Activation of filopodia on one side of the growth cone leads to a concentration gradient of calcium across the growth cone, providing a possible basis for changes in the direction of growth.
Other second messengers that link receptors and motor molecules include cyclic nucleotides. These nucleotide second messengers modulate the activity of enzymes such as protein kinases, protein phosphatases, and rho-family guanosine triphosphatases (GTPases). In turn these messengers and enzymes regulate the activity of proteins that regulate the polymerization and depolymerization of actin filaments, thereby promoting or inhibiting axonal extension.
The critical role of intracellular signals in growth cone motility and orientation can be demonstrated using embryonic neurons grown in culture. Application of growth factors to one side of a growth cone activates receptors locally and leads to extension and turning of the growth cone toward the source of the signal. In essence, the factor attracts the growth cone. Yet when cyclic adenosine monophosphate (cAMP), levels in the neuron are decreased, the same stimulus acts as a repellent and the growth cone turns away from the signal (Figure 54-7). Other repulsive factors can become attractive when levels of the second messenger cyclic guanosine 3’-5’-monophosphate (cGMP) are raised.
Figure 54-7 Changes in the level of intracellular regulatory proteins can determine whether the same extrinsic cue attracts or repels the growth cone. The state of protein kinase A (PKA) activity can alter the growth cone’s response to an extracellular orienting factor, in this instance the protein netrin. When PKA activity and intracellular cAMP levels are low, the growth cone is repelled by netrin. When PKA activity is high, the resulting elevation in intracellular cAMP causes the growth cone to be attracted to a local source of netrin. (Adapted, with permission, from Ming et al. 1997.)
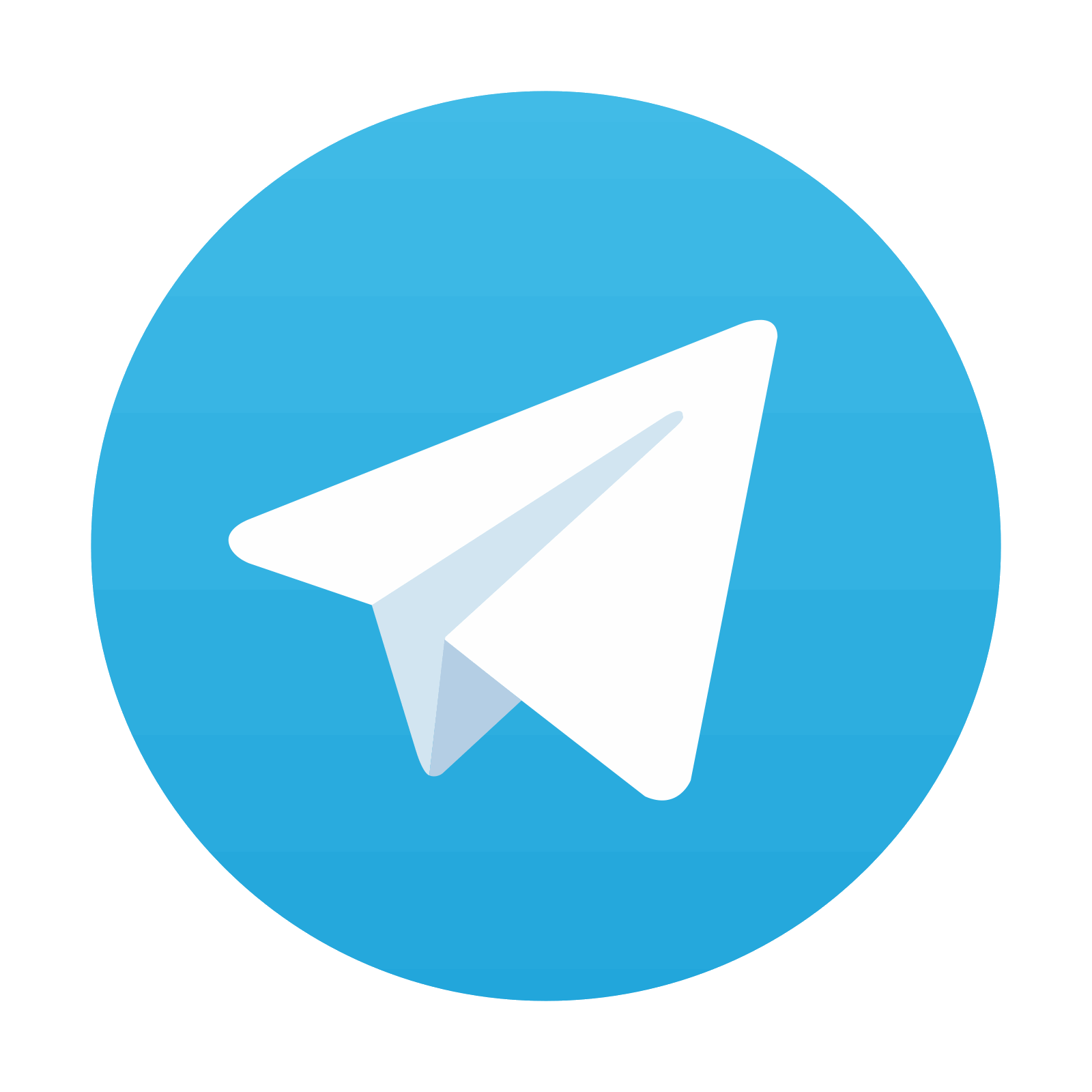
Stay updated, free articles. Join our Telegram channel
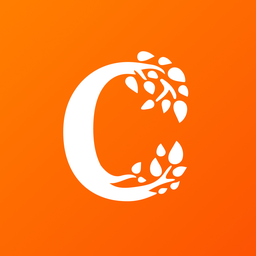
Full access? Get Clinical Tree
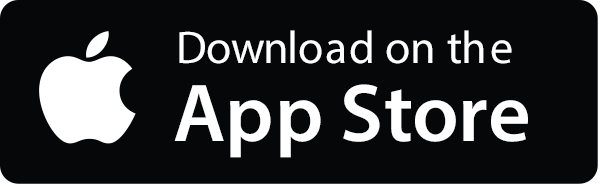
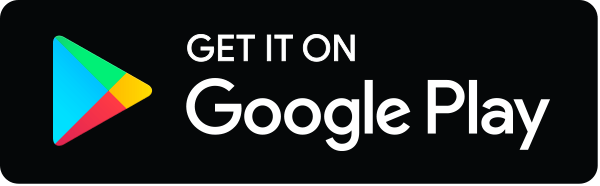