X-ray attenuation (HU)
Gray matter
35–45
White matter
20–30
Cerebro-spinal-fluid
4–8
Skull
100–1,000
Large vessels
40–50
Tissue calcification
80–150
Hematoma
70–90
Fat
−60 to −70
Air
−1,000
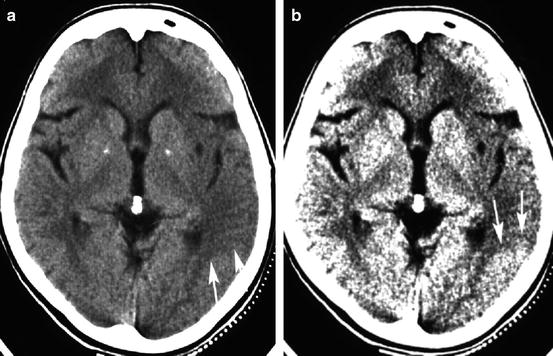
Fig. 12.1
Two representations of the identical section of a CT in a patient with acute stroke. Both sections are displayed with the same level, but with a different window width. With a window width of 80 HU (Panel a), the contrast between normal cortical density and cortical hypodensity is less obvious than with a window width of 31 HU (Panel b). The area of hypodensity covers part of the temporal lobe, the entire insular cortex, and the lateral rim of the putamen and is better outlined with the narrow CT window (arrows). Note the increase of noise in Panel b
The electron densities of the substrate under study attenuate x-rays [3]. In biological tissue, x-ray attenuation is directly correlated with the tissue specific gravity [4]. The different electron densities of gray and white matter, brain vessels, cerebro-spinal fluid (CSF), and skull allow to differentiate these structures on CT and to recognize pathological alterations.
Technical Note: How to Perform CT in Acute Stroke
If the imaging facility is informed in advance, the scanner can be kept free for the patient with acute stroke. Emergent life support should be continued during the patient’s imaging test if necessary. Direct transportation of the patient to the scanner will save time. Neurological examination can be performed on the scanner. While a detailed neurologic examination is not needed before the brain imaging is done, localizing the stroke to the posterior fossa or the cerebral hemisphere will assist in optimizing the imaging techniques selected.
CT should be first performed without contrast with a rapid scan time to reduce motion artifact. A correct head position is crucial to avoid obliquity of the sections. If necessary due to motion artifact, specific section cuts can be repeated. The high density of bone often causes an artifact, which may impair visualization of the lower part of the brain stem in the posterior fossa. Single slice scanning should be preferred to continuous spiral scanning. Thin (e.g. 0.625 mm) transaxial sections with 2.5 mm and 5 mm reconstructions can minimize artifacts and enhances sensitivity for thrombus detection [5]. Image windows should be adjusted so that gray and white matter can be easily distinguished and subtle hypodensities are detected. A window width of 70–80 HU at a center of 35–40 HU is recommended. A close communication between the stroke physician and the radiologists or technician performing the scan will enhance the information gained from CT. The image should be optimized for spatial and contrast resolution in the region of interest. Additional bone window views can be performed if head trauma is a possibility, in order to search for skull fractures, subdural air or blood, or effusions in nasal sinuses and middle ear. A contrast-enhanced scan might be obtained after, if there is suspicion for neoplasm or localized infection and no MRI available.
In case of suspected major cerebral artery occlusion that may require intra-arterial intervention, CT angiography (CTA) should be prepared and performed immediately after non-enhanced CT. Immediate interpretation of the non-enhanced scan may allow the start of thrombolytic infusion in parallel to CTA.
Advanced CT technology provides the opportunity of CTA and CT perfusion imaging with one contrast injection only [6, 7]. A conventional CTA is performed after bolus injection of 130 ml non-ionic contrast (injection rate: 4–5 ml/s) with a spiral scan of the brain base and 3-dimensional reconstruction of the Circle of Willis on a workstation. Perfusion imaging with CT requires repeated imaging of one or more sections to measure the contrast uptake and clearance curve in each voxel. Parameter images are then calculated for cerebral blood volume (CBV), mean transit times (MTT), cerebral blood flow (CBF), or time intervals to the peaks of contrast enhancement—time to peak (TTP) (Fig. 12.2) [8]. Multidetector CT technique now allows whole brain perfusion imaging.
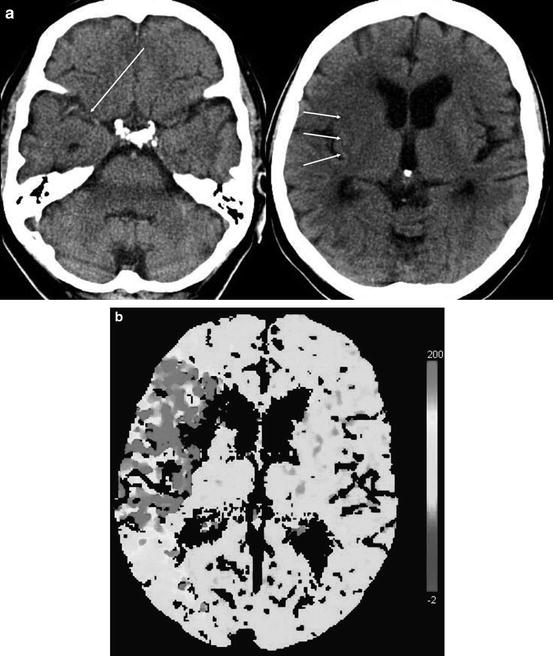
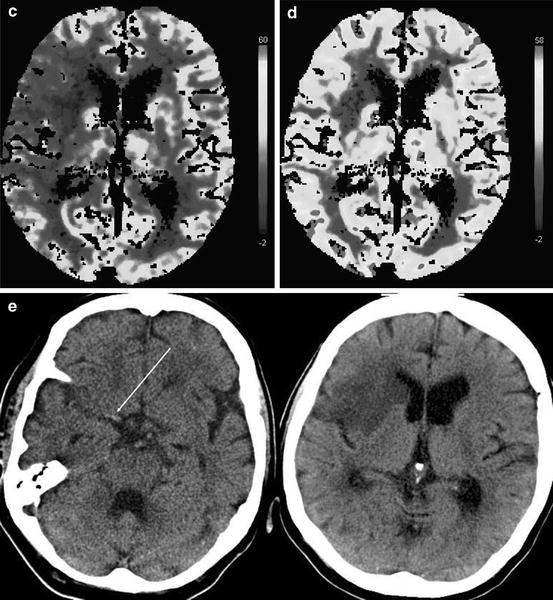
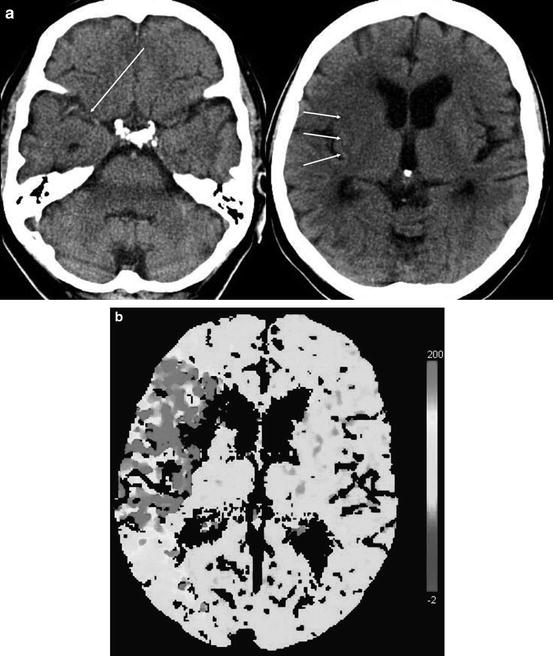
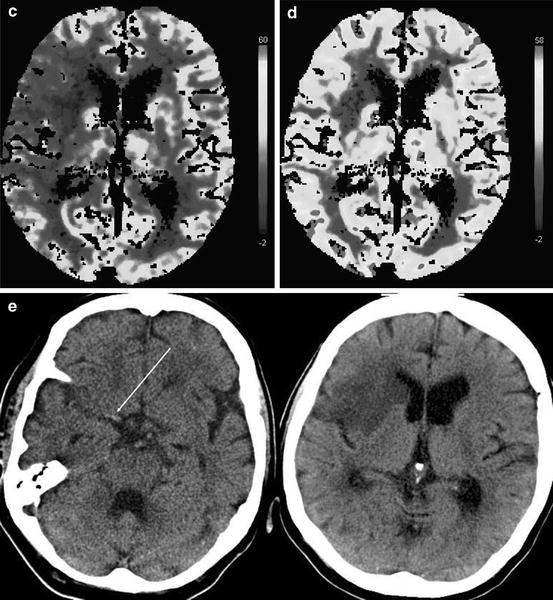
Fig. 12.2
CT and perfusion CT in an 82-year-old woman 2 h after the onset of a left-sided hemiparesis. The right MCA trunk is occluded (Panel a, long arrow) and the putamen and the frontal insular cortex are hypodense (Panel a, short arrows). The time-to-peak-map (TTP, Panel b) shows an area of delayed contrast inflow exceeding the hypoattenuating volume of brain tissue. No contrast peak could be identified within the right striatum due to very low blood flow. The cerebral blood flow (CBF, Panel c) map shows diminished flow of the area indicated by the TTP map, pronounced in the right striatum and insular cortex. Cerebral blood volume (CBV, Panel d) is diminished only within the right striatum and insular cortex. Despite thrombolysis, the right MCA remained occluded (Panel e, long arrow) as shown on the follow-up CT 1 day later. The infarct covers exactly the tissue volume that was hypodense on the baseline CT and identified by low CBV. The TTP- and CBF-maps showed a volume of brain tissue with disturbed perfusion that did not convert into infarct until day 1 after stroke onset
Interpretation of CT Findings
Pathological findings on non-enhanced CT (NCT) in acute stroke patients may be thrombo-embolic occlusion of large vessels, focal brain tissue swelling caused by vasodilatation, ischemic edema, intracranial hemorrhage, focal brain tissue inflammation, or tumor like lesions. Computed tomography identifies these findings by detecting changes in x-ray attenuation of normal brain structures, a shift or replacement of brain structures by pathological substrates, or pathological contrast enhancement. A pathological increase in x-ray attenuation is called “hyperdensity”, a pathological decrease “hypodensity”. These terms are somewhat confusing because they do not define a fixed degree of x-ray attenuation. They are commonly used to characterize the attenuation of a structure in comparison to other tissue, e.g. in saying that a parenchymal hematoma is identified by its hyperdensity if compared with gray matter. These terms are best used, however, to characterize a change in x-ray attenuation by comparing the “density” of an affected structure to its normal “density”. The symmetry of the brain structures in transaxial planes facilitates this comparison. e.g., the putamen is best evaluated by comparing its attenuation to that of the contralateral putamen and to that of the head of the caudate nucleus, because the caudate nucleus and the putamen are portions of the same anatomical structure, the striatum (Fig. 12.3). It is obvious, that low technical quality of the scan (motion artifacts, wrong window width or wrong level) and in particular any obliquity of the scan impair the recognition of real changes in x-ray attenuation (Fig. 12.4).
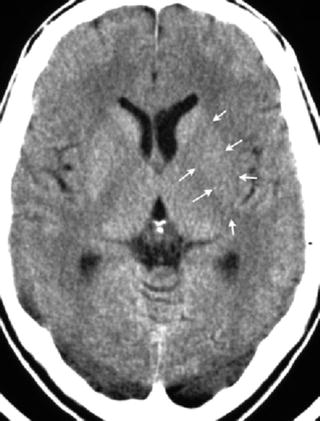
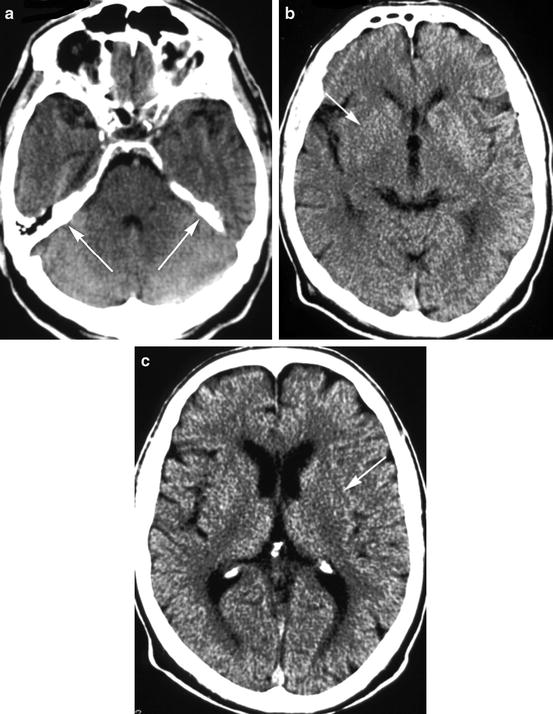
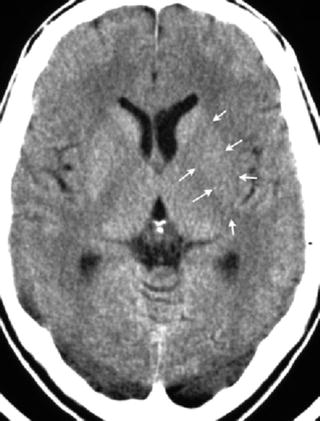
Fig. 12.3
Hypodensity of the left lentiform nucleus (arrows). X-ray attenuation is here less in comparison to the right lentiform nucleus, and attenuation of the left putamen is less than the attenuation of the left head of caudate nucleus. With further decline in attenuation, the lentiform nucleus cannot be distinguished from the internal and external capsule; it will be obscured
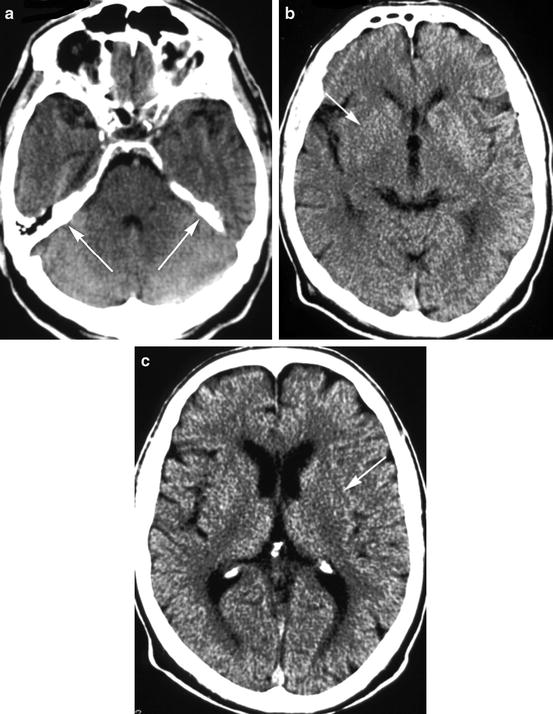
Fig. 12.4
The obliquity of a CT scan can be recognized by comparing the upper rim of both pyramids (arrows) (Panel a). In consequence of this obliquity, the right lentiform nucleus appeared less dense in one section (Panel b), and the left putamen appeared less dense in the adjacent upper section when compared to the contralateral side (Panel c)
The Detection of Stroke Pathology by CT
Clot Imaging
Thrombo-embolic occlusion of large brain arteries may result in a segmental hyperdensity of the artery (Fig. 12.2a, e). This finding is called “hyperdense artery sign” and is highly specific for the obstruction of this artery, if “hyperdensity” is defined as an increased x-ray attenuation of one arterial segment with various length in comparison to other portions of the same artery or its contra-lateral counterpart [9]. A hyperdense MCA trunk was observed in 48 % of patients with angiographically proven MCA trunk occlusion by applying 5 mm thick slices [10]. Previous work suggested that the hematocrit of thrombi affects x-ray attenuation and thus the CT detection sensitivity [11]: a red thrombus—relatively higher red blood cell content—would be detected more often than a white thrombus—fewer red blood cells—on CT. More recently, it was demonstrated that the sensitivity of non-enhanced CT for thrombus detection can be enhanced on multi-detector scanners up to 90 % with a collimation of 16 × 0.625 mm and reconstructions of 0.625 mm thick slices being converted into 5 mm thick slices using maximum intensity projections [5].
A hyperdense artery is not an “infarct sign”, because arteries can occlude without a subsequent or immediate brain infarct due to relatively preserved collateral blood supply. A hyperdense MCA trunk in association with normal tissue density may indicate a large tissue volume at risk from hypoperfusion, but no irreversible damage. Nevertheless, a hyperdense MCA trunk is often associated with a severe stroke and large infarct [12]. It is, therefore, prudent to carefully examine the territory of a hyperdense artery for parenchymal hypodensity.
Edema Imaging
Brain ischemia can cause three different types of edema: cytotoxic or cellular, ionic, and vasogenic [13]. The cytotoxic edema is the consequence of ion pump failure and influx of Na+-ions and water molecules into neurons following the Starling principle. Cytotoxic edema means a shift of water from the extracellular space into the cellular space with extension of the cells and shrinkage of the extracellular compartment. This water shift does not affect x-ray attenuation of the tissue, but affects the diffusibility of protons. Cytotoxic edema is thus not detectable by CT, but by diffusion weighted MRI (DWI).
The ionic edema is the replacement of water in the extracellular space in case of any capillary rest flow and means net water uptake by the ischemic brain tissue and consequently its change of x-ray attenuation. It was shown that brain tissue water content increases immediately after experimental MCA occlusion [14]. This occurs only in brain tissue suffering from CBF below 10 ml/100 g × min.
The vasogenic edema is a consequence of major blood-brain-barrier break down with extravasation of macromolecules and bulk water causing mass effects. This occurs very seldom within the first 12 h of stroke onset, is a consequence of reperfusion, and may lead to the shift of midline structures and temporal lobe herniation with midbrain compression thus requiring decompressive surgery.
In addition to ionic and vasogenic edema, reactive vasodilatation can cause brain parenchyma swelling. Brain swelling due to vasodilatation can develop under two conditions: 1) with low perfusion pressure, but intact cerebrovascular autoregulatory capacity, and 2) with venous obstruction [15, 16]. This type of brain tissue swelling is not associated with hypodensity, but can be associated with hyperdensity because of the increase in regional CBV. Brain swelling with iso- or hyperdensity is reversible, if the arterial or venous obstruction is treated successfully.
It could be difficult detecting subtle brain swelling on CT early after ischemia onset. In some cases early swelling can be inferred because enlargement of brain tissue diminishes the CSF space. Asymmetric loss of CSF spaces (“effacement”) might imply early tissue swelling, but because of the natural asymmetry of cerebral sulci, cisterns, and ventricles, it could be hard to decide whether the CSF space is unilaterally compressed or contra laterally enlarged. Clearer signs are the regional effacement of sulci, compression of the lateral ventricle, and in particular the combination of both (Fig. 12.5).
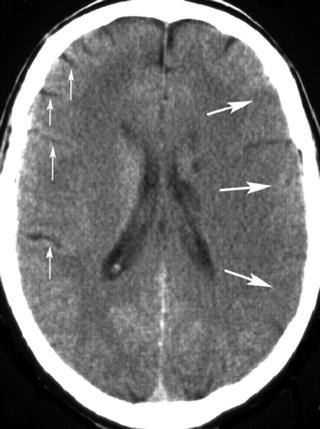
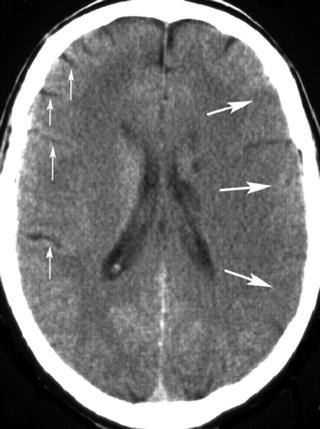
Fig. 12.5
CT scan obtained in a 40-year-old man with severe right sided hemiparesis. Effacement of the left cortical sulci in addition to hypodensity (large arrows). For comparison note the sulci of the right cerebral hemisphere (small arrows)
A decline in CBF below 10 ml/100 g × min causes the brain tissue to immediately take up water [17, 18]. In experimental animals, tissue water concentration increased steadily from 80.7 to 83 % within 4 h of middle cerebral artery (MCA) occlusion [19]. An increase by 1 % of tissue water content causes a decrease of x-ray attenuation by 2–3 HU [20]. In own experiments, x-ray attenuation declined by 7.5 ± 1.6 HU within 4 h of MCA occlusion and hypodensity became visible in the MCA territory of 2 of 10 animals within 2 h after MCA occlusion. Hypodensity could be differentiated from normal brain parenchyma in all ten animals by 3 h after MCA occlusion [21]. The decline in attenuation at 4 h by 7.5 ± 1.6 HU corresponds to a 2.5–3.8 % increase in brain tissue water content in good agreement with the observations by Schuier and Hossmann [17]. Moreover, we could show that x-ray attenuation correlates inversely with the degree of ischemic edema [21]. Using a CT window of 80 HU or less, the contrast resolution is 4 HU or less which corresponds with an increase in brain tissue water content of <2 %. Hypodensity of gray matter causes a diminished contrast to adjacent white matter and thus a loss of anatomical margins. Gray matter hypodensity, thus explains negative phenomena like “obscuration of lentiform nucleus” [22] and “loss of the insular ribbon” [23], so called “early infarct signs” (Figs. 12.2 and 12.4). Gray matter hypodensity in its early stage causes a loss in anatomical information, which may explain why this finding is easily missed.
The delayed detection of parenchymal hypodensity after arterial occlusion has another important consequence: The CT finding of parenchymal hypodensity is highly specific for irreversible tissue damage, because ischemic edema becomes irreversible within 2 h [17, 24] and indicates irreversibly damaged brain tissue [18]. A CT scan without hypodensity, however, implies that at least some reversible ischemic edema may be present, and entirely irreversible ischemic tissue damage has not developed.
In contrast to the subtle changes on the CT gray scale, diffusion weighted MR imaging (DWI) shows ischemic brain parenchyma with high signal that is easily discernible from normal brain tissue. Both imaging modalities do not show the same brain pathology, however. The reduction of cerebral blood flow (CBF) down to 30 ml/100 g × min causes cell swelling and the reduction of the extracellular fluid space [17]. Exactly at this CBF value, the apparent diffusion coefficient (ADC) of protons start to decline [25, 26]. Diffusion weighted MRI is thus sensitive for much milder degrees of brain ischemia than CT. The critical CBF threshold for signal increase on DWI is even above the CBF threshold for neuronal dysfunction, whereas CT hypodensity indicates ischemic tissue damage. In other words: brain tissue with disturbed proton diffusion can recover, but brain tissue with diminished x–ray attenuation cannot recover if blood flow is restored.
Partial volume artifacts may mimic parenchymal hypodensity on CT. These artifacts occur if the CT section is in parallel to the brain’s surface and includes parenchyma and CSF in same voxels. A typical location for this artifact is the temporal lobe.
Because hypoattenuating brain tissue represents ischemic edema and irreversible injury, its extent and location might be associated with the patient’s prognosis and response to reperfusion therapy. The ECASS group, therefore, suggested excluding patients with hypoattenuating brain tissue exceeding 1/3 of the MCA territory from the first trial of recombinant tissue plasminogen activator (rt-PA) for stroke. Another approach is to subdivide the MCA territory into ten regions and to subtract 1 point for each region being affected by ischemic edema. The Alberta Stroke Program Early CT Score (ASPECTS) thus provides information about the extent, pattern, and location of ischemic edema [27]. It was shown that rt-PA treated stroke patients have an almost 90 % risk to remain dependent with hypodensity on CT exceeding 1/3 of MCA-territory or an ASPECTS of 7 and lower. A retrospective analysis of PROACT data showed a risk reduction by intraarterial pro-Urokinase treatment only in patients with MCA-trunk occlusions and an ASPECTS >7 [28].
The Detection of Intracranial Hemorrhage and Stroke Mimics by CT
In acute stroke, blood may be present in one or more of cranial compartments: brain parenchyma, ventricles, subarachnoid space, and subdural or epidural space. Clinically, an acute parenchymal hemorrhage cannot be distinguished reliably from ischemic stroke. After acute hemorrhage, blood appears as a hyperattenuated, often space-occupying mass if clotted (Fig. 12.6). The degree of hyperdensity depends on the amount of blood, its hematocrit, whether it is clotted or not, and whether the blood is intermixed with CSF or brain tissue. Hemorrhages related to coagulopathies or treatment with anticoagulants or thrombolytics are often inhomogeneous with fluid levels (Fig. 12.7). Sensitivity of CT for the detection of parenchymal hemorrhage is nearly 100 %, but small hemorrhages into the brain parenchyma or subarachnoid space can be overlooked (Fig. 12.8) in particular if the patient has a low hematocrit, e.g., in hematologic disorders. The investigators of the European Cooperative Acute Stroke Studies (ECASS, ECASS II) missed two small parenchymal hemorrhages and one subarachnoid hemorrhage (SAH) in 1,420 patients (0.1 %) [29, 30]. The detection of blood within infarcted, hypodense brain tissue is problematic, but of importance. The hemorrhagic transformation of ischemic brain tissue is normal; the extent of hemorrhage varies under the influence of reperfusion, thrombolytics, and anticoagulants. Hemorrhagic transformation may appear on CT like normal gray matter surrounded by ischemic edema—slightly hyperattenuating spots scattered within the infarct—or it may present as a dense hematoma within the ischemic edema, with and without space occupying effect. It is unlikely that mild degrees of hemorrhagic transformation affect the neurological status of the patient. The term “symptomatic hemorrhage” for each sign of blood within ischemic infarctions is, therefore, highly problematic and may have contributed to the overestimation of the risk from these types of bleedings after thrombolytic therapy [31]. It was shown that only intracerebral hematomas with space occupying effect are associated with clinical deterioration and poor outcome [32, 33]. The ECASS investigators have used categories for four different types of hemorrhagic transformation of ischemic brain tissue: hemorrhagic infarction, grade 1 and 2 (HI1 and HI2), and parenchymal hematoma, grade 1 and 2 (PH1 and PH2) [34]. The location of hematoma may provide clues about its underlying etiology (Table 12.2). Acute hemorrhages usually show hyperattenuation without surrounding edema. If marked edema is present under those circumstances, underlying neoplasm should be suspected. Multiple hemorrhagic lesions should suggest metastatic disease, coagulopathy, cavernomas, or cerebral amyloid angiopathy.
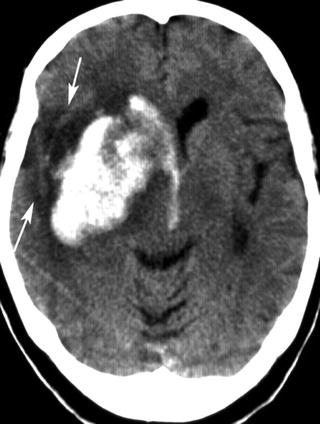
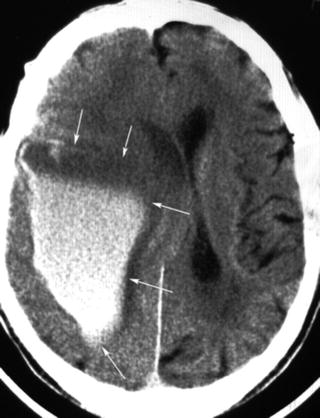
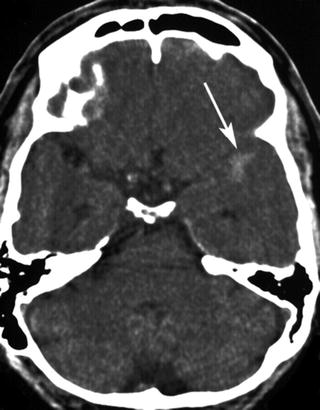
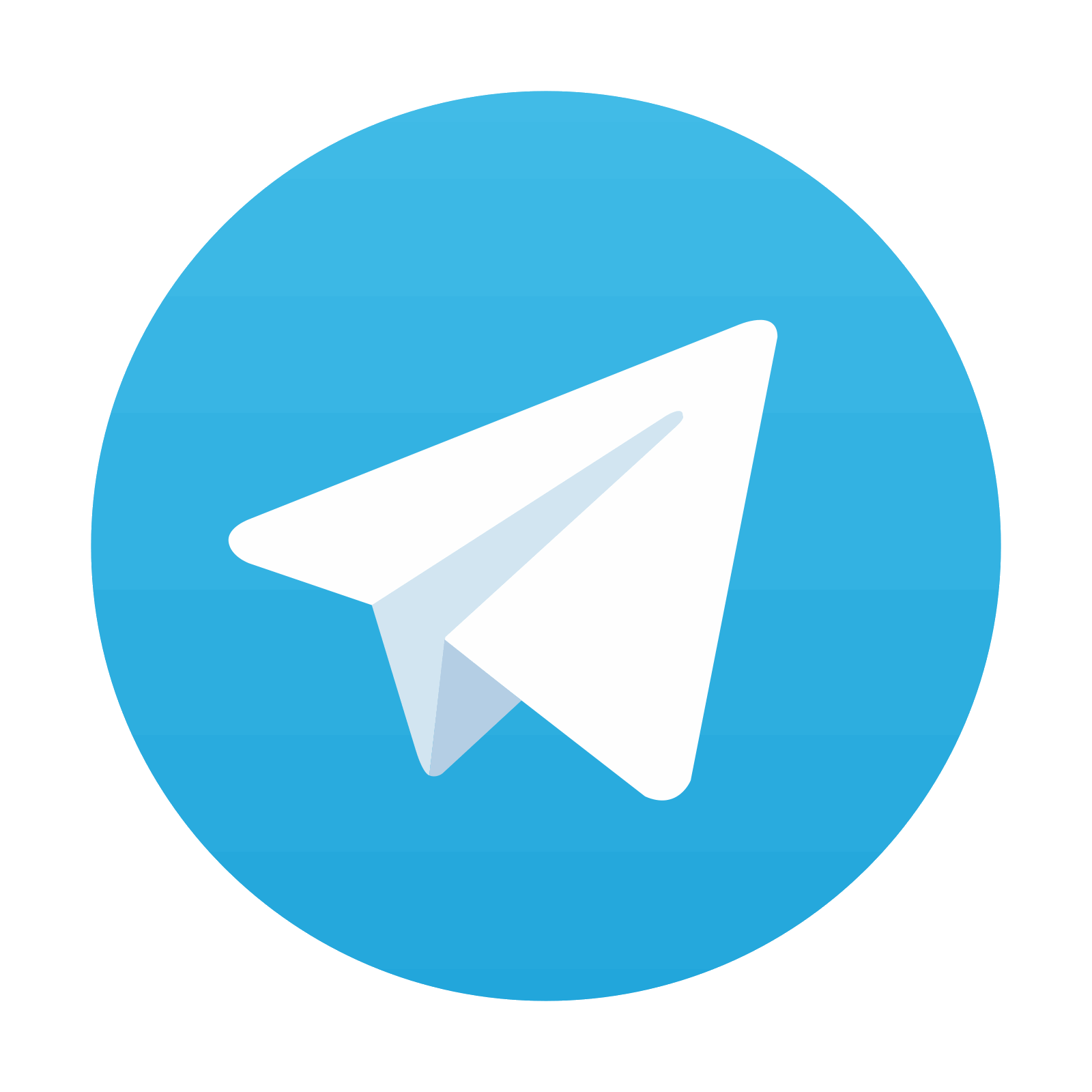
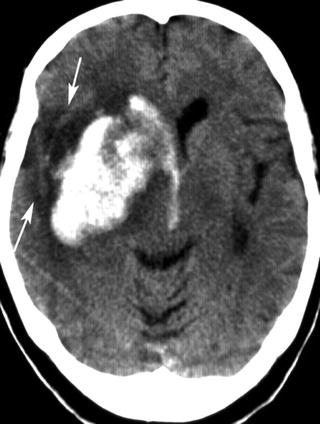
Fig. 12.6
Parenchymal hematoma of the right basal ganglia and intraventricular hemorrhage. The wedge shaped hypodensity lateral of the hematoma (arrows) is suspicious for an underlying ischemic infarction
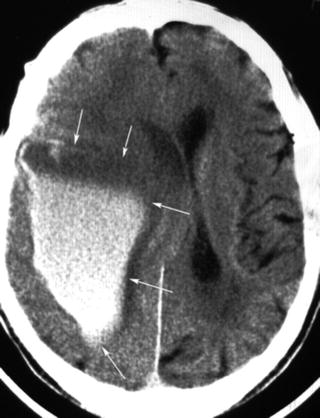
Fig. 12.7
Parenchymal hemorrhage after myocardial infarction and thrombolysis. Signs of unclotted blood: The upper portion is free of cells and hypodense. Increasing density in the lower portion due to sedimentation of cellular elements (arrows)
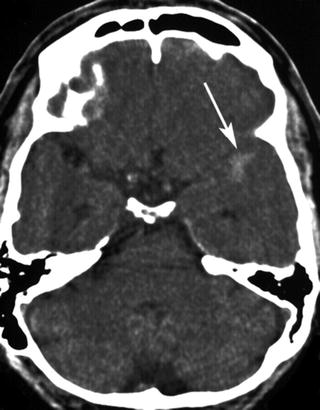
Fig. 12.8
Small hyperattenuated area in the left anterior Sylvian fissure (arrow). Low contrast because of broad window of 160 HU. The subarachnoidal hemorrhage was overlooked, and the patient was randomized to rt-PA. The hemorrhage was confirmed by the follow-up CT. The patient had an excellent clinical outcome
Table 12.2
Common causes of spontaneous cerebral hemorrhage and their common locations
Cause | Typical location |
---|---|
Arterial diseases | |
Microvessel disease | Basal ganglia, hemispheric white matter |
Amyloidangiopathy | Cortical-white matter junction |
Moya-moya | Basal ganglia |
Vasculitis | All territories, multiple |
Reversible vasoconstriction | Occipital, parietal |
Hemorrhagic transformation | All territories |
Aneurysms | Base of brain, corpus callosum |
Arterio-venous Malformation | All territories, ventricle wall |
Pericapillary disease | Cerebral white matter |
Venous malformations | All territories, even extra-axial |
Multiple locations | |
Conditions | |
Hypertension | Basal ganglia, external capsule |
Coagulopathy | All territories |
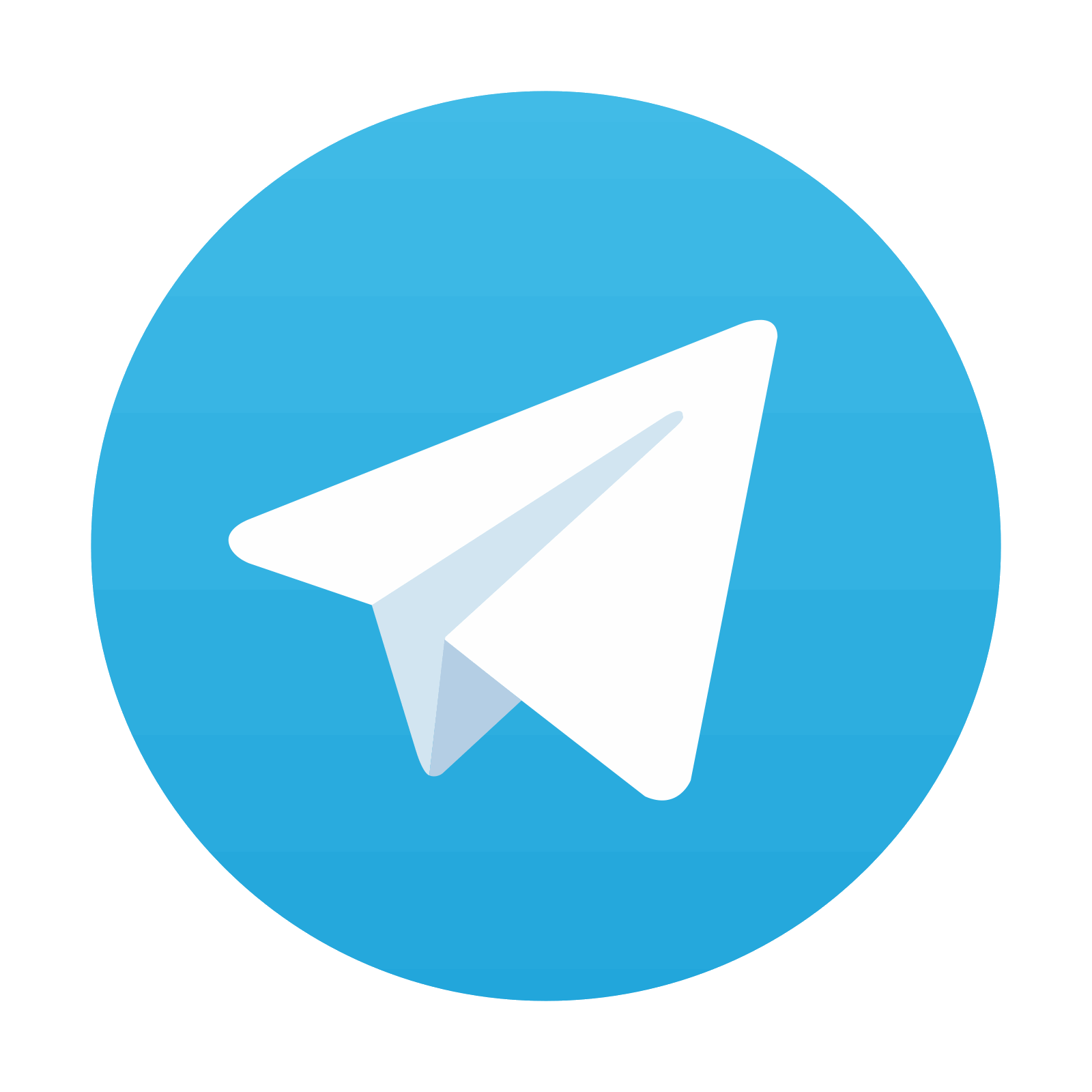
Stay updated, free articles. Join our Telegram channel
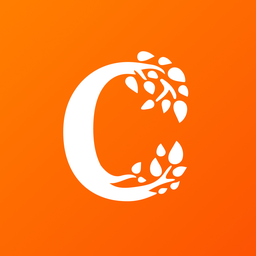
Full access? Get Clinical Tree
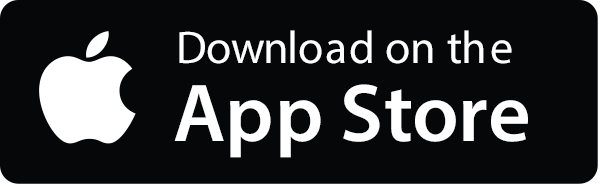
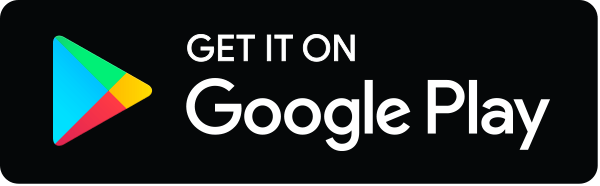