The Inner Ear
The Ear Has Three Functional Parts
Hearing Commences with the Capture of Sound Energy by the Ear
The Basilar Membrane Is a Mechanical Analyzer of Sound Frequency
The Organ of Corti Is the Site of Mechanoelectrical Transduction in the Cochlea
Hair Cells Transform Mechanical Energy into Neural Signals
Deflection of the Hair Bundle Initiates Mechanoelectrical Transduction
The Temporal Responsiveness of Hair Cells Determines Their Sensitivity
Hair Cells Use Specialized Ribbon Synapses
Auditory Information Flows Initially Through the Cochlear Nerve
Bipolar Neurons in the Spiral Ganglion Innervate Cochlear Hair Cells
Cochlear Nerve Fibers Encode Stimulus Frequency and Intensity
Sensorineural Hearing Loss Is Common but Treatable
HUMAN EXPERIENCE IS ENRICHED by our ability to distinguish a remarkable range of sounds—from the intimacy of a whisper to the warmth of a conversation, from the complexity of a symphony to the roar of a stadium. Hearing begins when the cochlea, the snail-shaped receptor organ of the inner ear, transduces sound energy into electrical signals and forwards them to the brain. Our ability to recognize small differences in sounds stems from the cochlea’s capacity to distinguish among frequency components and to inform us of both the tones present and their amplitudes.
Deafness can be devastating. For the elderly, hearing loss can result in a painful and protracted estrangement from family, friends, and colleagues. Children may lack hearing as a result of pre- or perinatal infections and especially of genetic conditions, which affect one child in a thousand. Such children are often deprived of the normal avenue to the development of speech, and thus of reading and writing as well. It is for this reason that a modern pediatric examination must include an assessment of hearing. Many children thought to be cognitively impaired are found instead to be hard of hearing, and their intellectual development resumes its normal course when this problem is corrected.
Acute hearing loss in the intermediate years exacts an enormous price for two reasons. First, hearing plays an important, but often overlooked, role in our psychological well-being. Daily conversation with family and colleagues helps to establish our social context. The abrupt loss of such social intercourse as a result of sudden deafness leaves a person distressingly lonely and may lead to depression and even suicide. Hearing also serves us in another, more subtle way. Our auditory system is a remarkably efficient early warning system, subconsciously informing us about our environment. For example, when other people enter the room or approach us we often hear them before we see them. More obviously, awareness of fire alarms and the sirens of emergency vehicles can be lifesaving. Deafness may leave a person with an ominous sense of vulnerability to unheard changes in the environment.
Hearing loss is often accompanied by another distressing symptom, tinnitus, or ringing in the ears. By interfering with concentration and disrupting sleep, tinnitus can exasperate, depress, and even madden its victims. Because on rare occasions tinnitus stems from lesions to the auditory pathways, such as acoustic neuromas, it is important in neurological diagnosis to exclude such causes. Most tinnitus, however, is idiopathic: Its cause is uncertain. Some drugs trigger the condition; antimalarial drugs related to quinine and aspirin at the high dosages used in the treatment of rheumatoid arthritis are notorious for this. Often, however, tinnitus occurs at high frequencies to which a damaged ear is no longer sensitive. In these instances tinnitus may reflect hypersensitivity in the deafferented central nervous system, a phenomenon analogous to phantom-limb pain (see Chapter 24).
Hearing depends on the remarkable properties of hair cells, the receptors of the internal ear. Hair cells receive mechanical inputs that correspond to sounds and transduce these signals into electrical responses that are forwarded to the brain for interpretation. These cells can measure motions of atomic dimensions and transduce stimuli ranging from static inputs to those at frequencies of tens of kilohertz. Hair cells also serve as mechanical amplifiers that augment our auditory sensitivity. Each of the paired cochleas contains approximately 16,000 of these cells. Deterioration of hair cells accounts for most of the hearing loss that afflicts more than 30 million Americans.
The Ear Has Three Functional Parts
Sound consists of alternating compressions and rarefactions propagated by an elastic medium, the air, at a speed of approximately 340 m/s. As we are reminded on making the effort to shout, producing these pressure changes requires that work be done on the air by our vocal apparatus or some other sound source. Each of our ears must capture this mechanical energy, transmit it to the receptor organ, and transduce it into electrical signals suitable for neural analysis. These three tasks are the functions of, respectively, the external ear, the middle ear, and the inner ear (Figure 30–1).
Figure 30-1 The structure of the human ear. The external ear, especially the prominent auricle, focuses sound into the external auditory meatus. Alternating increases and decreases in air pressure vibrate the tympanum. These vibrations are conveyed across the air-filled middle ear by three tiny, linked bones: the malleus, the incus, and the stapes. Vibration of the stapes stimulates the cochlea, the hearing organ of the inner ear.
The most obvious component of the human external ear is the auricle, a prominent fold of cartilage-supported skin. Much as a parabolic antenna collects electromagnetic radiation, the auricle acts as a reflector to capture sound efficiently and to focus it into the external auditory meatus, or ear canal. The external auditory meatus ends at the tympanum or eardrum, a thin diaphragm approximately 9 mm in diameter.
The external ear is not uniformly effective at capturing sound from any direction; the auricle’s corrugated surface collects sounds best when they originate at different, but specific, positions with respect to the head. Our capacity to localize sounds in space, especially along the vertical axis, depends critically on the sound-gathering properties of the external ear.
The middle ear is an air-filled pouch connected to the pharynx by the Eustachian tube. Airborne sound traverses the middle ear as vibrations of three tiny ossicles, or bones: the malleus (hammer), incus (anvil), and stapes (stirrup). The base of the malleus is attached to the tympanic membrane; its other extreme makes a ligamentous connection to the incus, which is similarly connected to the stapes. The flattened termination of the stapes, the footplate, inserts in an opening—the oval window—in the bony covering of the cochlea. The first two ossicles are relics of evolution, for their antecedents served as components of the jaw in reptilian ancestors.
The inner ear, or cochlea (from the Greek cochlos, meaning snail), is a coiled structure of progressively diminishing diameter wound like a snail’s shell around a conical bony core (Figure 30–1). It is approximately 9 mm across, the size of a chickpea. The cochlea is covered with a thin layer of laminar bone and embedded within the dense structure of the temporal bone. The inner and outer aspects of the cochlea’s bony surface are lined with layers of connective tissue, the endosteum and periosteum.
The interior of the cochlea consists of three liquid-filled compartments termed scalae (Latin, meaning staircases). In a cross section of the cochlea at any position along its spiral course, the compartment farthest from the base is the scala vestibuli (Figure 30–2). At the basal end of this chamber is the oval window, an opening that is sealed by the footplate of the stapes. The chamber nearest the cochlear base is the scala tympani; it too has a basal aperture, the round window, which is closed by a thin, elastic diaphragm. The two chambers are separated along most of their length by the cochlear partition or duct. The scala vestibuli and scala tympani communicate with one another at the helicotrema, where the cochlear partition terminates slightly below the cochlear apex.
Figure 30-2 The structure of the cochlea. A cross section of the cochlea shows the arrangement of the three liquid-filled ducts or scalae, each of which is approximately 33 mm long. The scala vestibuli and scala tympani communicate through the helicotrema at the apex of the cochlea. At the base each duct is closed by a sealed aperture. The scala vestibuli is closed by the oval window, against which the stapes pushes in response to sound; the scala tympani is closed by the round window, a thin, flexible membrane. Between these two compartments lies the scala media, an endolymph-filled tube whose epithelial lining includes the 16,000 hair cells in the organ of Corti surmounting the basilar membrane. The cross section in the lower diagram has been rotated so that the cochlear apex is oriented toward the top.
The third liquid-filled cavity, the scala media, lies within the cochlear partition. A pair of elastic structures separates the scala media from the other ducts (Figure 30–2). The thin Reissner’s membrane, or vestibular membrane, divides the scala media from the scala vestibuli. The basilar membrane separates the scala media from the subjacent scala tympani; it is a complex structure involved in auditory transduction.
Hearing Commences with the Capture of Sound Energy by the Ear
Psychophysical experiments have established that we perceive an approximately equal increment in loudness for each tenfold increase in the amplitude of a sound stimulus. This type of relation is characteristic of many of our senses and is the basis of the Weber-Fechner law (see Chapter 21). A logarithmic scale is therefore useful in relating quantitatively sound intensity to perceived loudness. The sound-pressure level, L, of any sound may be expressed in decibels as
in which P, the magnitude of the stimulus, is the root-mean-square sound pressure (in units of pascals, abbreviated Pa, or newtons per square meter). For a sinusoidal stimulus the amplitude exceeds the root-mean-square value by a factor of √2. The reference level on this scale, 0 dB, corresponds to a root-mean-square sound pressure, PREF, of 20 µPa. This level represents the approximate threshold of human hearing at 1 to 4 kHz, the frequency range in which our ears are most sensitive.
That sound consists of alternating changes in the local air pressure is evident when a loud noise rattles a window. The loudest sound tolerable to humans, with an intensity of approximately 120 dB, transiently alters the local atmospheric pressure by only ±0.01%. Acting on a window 1 m on each side, this oscillatory pressure nonetheless produces a force of ±14 newtons. To rattle the same window by pushing upon it, we would need to exert a comparable force, approximately ±3 lb. In contrast, a tone at the threshold level causes a fractional change in the local pressure of much less than one part in a billion.
Despite their small magnitude, sound-induced increases and decreases in air pressure push and pull effectively upon the tympanum, moving it inward and outward (Figure 30–3A, B). The subsequent motions of the ossicles are complex, depending on both the frequency and the intensity of sound. In simple terms, however, the actions of these bones may be understood as those of two interconnected levers (the malleus and incus) and a piston (the stapes). The vibration of the incus alternately drives the stapes deeper into the oval window and retracts it. The footplate of the stapes thus serves as a piston that pushes and pulls cyclically upon the liquid in the scala vestibuli. The overall effect of the middle ear is to match the impedance of the air outside the ear to that of the cochlear partition, thus ensuring the efficient transfer of sound energy from the first medium to the second.
Figure 30-3 (Opposite) Motion of the basilar membrane.
A. An uncoiled cochlea, with its base displaced to show its relation to the scalae, indicates the flow of stimulus energy. Sound vibrates the tympanum, which sets the three ossicles of the middle ear in motion. The piston-like action of the stapes, a bone inserted partially into the elastic oval window, produces oscillatory pressure differences that rapidly propagate along the scala vestibuli and scala tympani. Low-frequency pressure differences are shunted through the helicotrema, where the two ducts communicate. The oval and round windows do not actually lie at the extreme base of the cochlea, but occur at oblique angles slightly toward the apex.
B. The functional properties of the cochlea are conceptually simplified if the cochlea is viewed as a linear structure with only two liquid-filled compartments separated by the elastic basilar membrane.
C. If the basilar membrane had uniform mechanical properties along its full extent, a compression would drive the tympanum and ossicles inward, increasing the pressure in the scala vestibuli and forcing the basilar membrane downward. Opposite movements would occur during a rarefaction. The pressure changes in the scala tympani are relieved by bowing of the round-window membrane. The movements of the tympanum, ossicles, and basilar membrane are greatly exaggerated.
D. In fact, the basilar membrane’s mechanical properties vary continuously along its length. The oscillatory stimulation of a sound causes a traveling wave on the basilar membrane, shown here within the envelope of maximal displacement over an entire cycle. The magnitude of movement is grossly exaggerated in the vertical direction; the loudest tolerable sounds move the basilar membrane by only ±150 nm, a scaled distance less than one-hundredth the width of the lines representing the basilar membrane in these figures.
E. An enlargement of the active region in D demonstrates the motion of the basilar membrane in response to stimulation with sound of a single frequency. The continuous curve depicts a traveling wave at one instant; the vertical scale of basilar-membrane deflection is exaggerated about one-millionfold. The dashed and dotted curves portray the traveling wave at successively later times as it progresses from the cochlear base (left) toward the apex (right). As the wave approaches the characteristic place for the stimulus frequency, it slows and grows in amplitude. The stimulus energy is then transferred to hair cells at the position of the wave’s peak.
F. Each frequency of stimulation excites maximal motion at a particular position along the basilar membrane. Low-frequency sounds produce basilar-membrane motion near the apex, where the membrane is relatively broad and flaccid. Mid-frequency sounds excite the membrane in its middle. The highest frequencies that we can hear excite the basilar membrane at its narrow, taut base. The mapping of sound frequency onto the basilar membrane is approximately logarithmic.
G. The basilar membrane performs spectral analysis of complex sounds. In this example a sound with three prominent frequencies, such as the three formants of a vowel sound, excites basilar-membrane motion in three regions, each of which represents a particular frequency. Hair cells in the corresponding positions transduce the basilar-membrane oscillations into receptor potentials, which in turn excite the nerve fibers that innervate these particular regions.
The action of the stapes at the oval window produces changes in pressure that propagate throughout the liquid of the scala vestibuli at the speed of sound. Because the aqueous perilymph is virtually incompressible, however, the primary effect of the stapes’s motion is to displace the liquid in the scala vestibuli in the one direction that is not restricted by a rigid boundary: toward the elastic cochlear partition (Figure 30–3B). The deflection of the cochlear partition downward increases the pressure in the scala tympani. The enhanced pressure displaces a liquid mass that causes outward bowing of the round window. Each cycle of a sound stimulus thus evokes a cycle of up-and-down movement of a minuscule volume of liquid in each of the inner ear’s three chambers.
Because the energy associated with acoustic signals is generally quite small, compromise of the middle ear’s normal structure may lead to conductive hearing loss, of which two forms are especially common. First, scar tissue caused by middle-ear infection (otitis media) can immobilize the tympanum or ossicles. Second, a proliferation of bone in the ligamentous attachments of the ossicles can deprive the ossicles of their normal freedom of motion. This chronic condition of unknown origin, termed otosclerosis, can lead to severe deafness.
A clinician may test for conductive hearing loss by the simple Rinné test. A patient is asked to compare the loudness of a vibrating tuning fork held in the air near an affected ear with that perceived when the base of the tuning fork is pressed against the patient’s head just behind the auricle. If the latter stimulus is perceived to be louder, the patient’s conductive pathway may be damaged but the internal ear is likely to be intact. In contrast, if bone conduction is not more efficient than airborne stimulation, the patient may have inner-ear damage, that is, sensorineural hearing loss. The diagnosis of conductive hearing loss is important because surgical intervention is highly effective: Removal of scar tissue or reconstitution of the conductive pathway with an inert prosthesis can in many instances restore excellent hearing.
The Hydrodynamic and Mechanical Apparatus of the Cochlea Delivers Mechanical Stimuli to the Receptor Cells
The Basilar Membrane Is a Mechanical Analyzer of Sound Frequency
The mechanical properties of the basilar membrane are key to the cochlea’s operation. To appreciate this, suppose that the basilar membrane had uniform dimensions and mechanical properties along its entire length, approximately 33 mm. Under these conditions a fluctuating pressure difference between the scala vestibuli and the scala tympani would move the entire basilar membrane up and down with similar excursions at all points (Figure 30–3C).
This would occur regardless of the frequency of stimulation; any pressure difference between the scala vestibuli and the scala tympani would propagate throughout those chambers within microseconds, so the basilar membrane would be subjected to similar forces at any position along its length. This simple form of basilar-membrane motion in fact occurs in the auditory organs of some reptiles.
In reality, however, the mechanical properties of the mammalian basilar membrane vary continuously along the cochlea’s length. The basilar membrane at the apex of the human cochlea is more than five times as broad as at the base. Thus, although the cochlear chambers become progressively larger from the organ’s apex toward its base, the basilar membrane decreases in width. Moreover, the basilar membrane is relatively thin and floppy at the apex of the cochlea but thicker and tauter toward the base. Because its mechanical properties vary along its length, the basilar membrane does not oscillate like a single string on a musical instrument; instead, it more resembles a panoply of strings that vary from the coarsest string on a bass viol to the finest string on a violin.
Stimulation with a pure tone evokes a complex and elegant movement of the basilar membrane. Over one complete cycle of a sound, each affected segment along the basilar membrane undergoes a single cycle of vibration (Figure 30–3D, E). The various parts of the membrane do not, however, oscillate in phase with one another; instead, some portions of the membrane move upward while others move downward. As first demonstrated by Georg von Békésy using stroboscopic illumination, the overall pattern of motion of the membrane is that of successive traveling waves.
Because mechanical stimulation is applied at the cochlear base as a pressure difference between scala vestibuli and scala tympani, the traveling wave progresses from the cochlear base toward the apex. Each wave reaches its maximal amplitude at a particular position appropriate for the frequency of stimulation, then declines rapidly in size as it advances toward the cochlear apex. A traveling wave ascending the basilar membrane resembles an ocean wave rolling toward the shore: As the wave nears the beach its crest grows to a maximal height, then breaks and rapidly fades away.
Although the analogy of an ocean wave gives some sense of the appearance of the basilar membrane’s motion, the connection between the cochlear traveling wave and the movement of an ocean wave is only metaphorical—the physical bases of the two waves are quite distinct. An ocean wave is the result of the momentum of a wind-blown mass of water. In contrast, movement of the basilar membrane is the result of motion of the liquid masses above and below the membrane. These liquids are continuously driven up and down by the energy supplied by the stapes’s piston-like movements at the oval window.
The variation in the mechanical properties of the mammalian basilar membrane explains why the membrane is tuned to different frequencies at each point along its length. In humans the membrane at the apex of the cochlea responds best to the lowest audible frequencies, down to approximately 20 Hz. At the cochlear base it responds to frequencies as great as 20 kHz. The intervening frequencies are represented along the basilar membrane in a continuous array (Figure 30–3F). In the 19th century the German physiologist Hermann von Helmholtz was the first to appreciate that the basilar membrane’s operation is essentially the inverse of a piano’s. The piano synthesizes a complex sound by combining the pure tones produced by numerous vibrating strings; the cochlea deconstructs a complex sound by isolating each component tone at a discrete segment of the basilar membrane.
The arrangement of vibration frequencies along the basilar membrane is an example of a tonotopic map. The relation between frequency and position on the basilar membrane varies smoothly and monotonically but is not linear. Instead, the logarithm of the best frequency is roughly proportional to the distance from the cochlea’s apex. The frequencies from 20 Hz to 200 Hz, those between 200 Hz and 2 kHz, and those spanning 2 kHz to 20 kHz are each apportioned approximately one-third of the basilar membrane’s extent.
Analysis of the response to a complex sound illustrates how the basilar membrane operates in daily life. A vowel sound in human speech, for example, ordinarily comprises three dominant frequency components termed formants. Measurement of the sound pressure outside an ear exposed to such a sound reveals a complex, seemingly chaotic signal. The movements of the tympanum and ossicles in response to a vowel sound likewise appear very complicated. The motion of the basilar membrane, however, is much simpler. Each frequency component of the stimulus establishes a traveling wave that, to a first approximation, is independent of the waves evoked by the others (Figure 30–3G). Each traveling wave reaches its peak excursion at a point on the basilar membrane appropriate for that frequency component. Moreover, the amplitude of each traveling wave is proportional, albeit in a complex way, to the intensity of the corresponding frequency component. The basilar membrane thus acts as a mechanical frequency analyzer by distributing specific stimulus energies to hair cells arrayed along its length, and in doing so begins the encoding of the frequencies and intensities in a sound.
The Organ of Corti Is the Site of Mechanoelectrical Transduction in the Cochlea
The organ of Corti, a ridge of epithelium extending along the basilar membrane, is the receptor organ of the inner ear. Each organ of Corti contains approximately 16,000 hair cells innervated by approximately 30,000 afferent nerve fibers, which carry information into the brain along the eighth cranial nerve. Like the basilar membrane itself, both the hair cells and the auditory nerve fibers are tonotopically organized: At any position along the basilar membrane the hair cells are most sensitive to a particular frequency, and these frequencies are logarithmically mapped in ascending order from the cochlea’s apex to its base.
The organ of Corti includes a variety of cells, many of obscure function, but four types have obvious importance. First, there are two varieties of hair cells. The inner hair cells form a single row of approximately 3,500 cells, whereas approximately 12,000 outer hair cells lie in three rows farther from the central axis of the cochlear spiral (Figure 30–4). The outer hair cells are supported at their bases by the Deiters’s (phalangeal) cells; the space between the inner and outer hair cells is delimited and mechanically supported by pillar cells.
Figure 30-4 Cellular architecture of the human organ of Corti. Although there are differences among species, the basic plan is similar for all mammals.
A. The organ of Corti, the inner ear’s receptor organ, is an epithelial strip that surmounts the elastic basilar membrane. The organ contains some 16,000 hair cells arrayed in four rows: a single row of inner hair cells and three rows of outer hair cells. The mechanically sensitive hair bundles of these receptor cells protrude into endo-lymph, the liquid within the scala media. Reissner’s membrane, which provides the upper boundary of the scala media, separates the endolymph from the perilymph in the scala vestibuli. The hair bundles of outer hair cells are attached at their tops to the lower surface of the tectorial membrane, a gelatinous shelf that extends the full length of the basilar membrane.
B. The hair cells are separated and supported by pillar cells and Deiters’s cells. One hair cell has been removed from the middle row of outer hair cells to reveal the three-dimensional relationship between supporting cells and hair cells. Efferent nerve endings at the bases of outer hair cells have been omitted from the drawing.
A second epithelial ridge adjacent to the organ of Corti, but nearer the cochlea’s central axis, gives rise to the tectorial membrane, a cantilevered gelatinous shelf that covers the organ of Corti (Figure 30–4). The tectorial membrane is anchored at its base by the interdental cells, which are also partly responsible for secreting this extracellular structure. The tectorial membrane’s tapered distal edge forms a fragile connection with the organ of Corti. When the basilar membrane vibrates in response to a sound, the organ of Corti and the overlying tectorial membrane move with it. Because the basilar and tectorial membranes pivot about different lines of insertion, their up-and-down motion is accompanied by back-and-forth shearing motion of the upper surface of the organ of Corti and the lower surface of the tectorial membrane. This motion is detected by hair cells.
Hair cells originate from the surface ectoderm of the embryo and retain an epithelial character. Columnar or flask-shaped, a hair cell lacks both dendrites and an axon (Figure 30–5A). Around its apex the hair cell is connected to nonsensory supporting cells. A special saline solution, the endolymph, bathes the cell’s apical aspect. A tight junction separates this liquid from the ordinary extracellular fluid, or perilymph, that contacts the basolateral surface of the cell. Immediately below the tight junction an intermediate junction provides a strong mechanical attachment for the hair cell.
Figure 30-5 Structure of a vertebrate hair cell.
A. The epithelial character of the hair cell is evident in this drawing of the sensory epithelium from a frog’s internal ear. The cylindrical hair cell is joined to adjacent supporting cells by a junctional complex around its apex. The hair bundle, a mechanically sensitive organelle, extends from the cell’s apical surface. The bundle comprises some 60 stereocilia arranged in stepped rows of varying length. At the bundle’s tall edge stands the single kinocilium, an axonemal structure with a bulbous swelling at its tip; in the mammalian cochlea this organelle degenerates around the time of birth. Deflection of the hair bundle’s top to the right depolarizes the hair cell; movement in the opposite direction elicits hyperpolarization. The hair cell is surrounded by supporting cells, whose apical surfaces bear a stubble of microvilli. Afferent and efferent synapses contact the basolateral surface of the plasma membrane.
B. This scanning electron micrograph of a hair cell’s apical surface reveals the hair bundle protruding approximately 8 μm into the endolymph.
The hair bundle, which serves as a receptor for mechanical stimuli, projects from the flattened apical surface of the hair cell. Cochlear hair bundles comprise a few hundred cylindrical processes, the stereocilia, arranged in a hexagonal array and extending several micrometers from the cell surface. Because successive stereocilia across a cell’s surface vary in length, a hair bundle is beveled like the tip of a hypodermic needle (Figure 30–5B). In the mammalian cochlea the inner hair cell bundles have a roughly linear cross-sectional form. Outer hair cell bundles, in contrast, have a V shape (Figure 30–6).
Figure 30-6 Arrangement of the hair cells in the organ of Corti.
A. Inner hair cells form a single row, and the stereocilia of each cell are arranged linearly. In contrast, outer hair cells are distributed in three rows, and the stereocilia of each cell are arranged in a V configuration. The apical surfaces of several other cells are visible: inner spiral sulcus cells, pillar cells, Deiters’s cells, and Hensen’s cells.
B. Higher magnification shows more clearly the V-shaped configuration of the hair bundle atop each outer hair cell. The hair-cell surface around the stereocilia is smooth, whereas the surfaces of supporting cells are endowed with microvilli.
Each stereocilium is a rigid cylinder whose cytoskeleton consists of a fascicle of actin filaments cross-linked by the proteins plastin (fimbrin), fascin, and espin. Cross-linking renders a stereocilium much more rigid than would be expected for a bundle of unconnected actin filaments. The core of the stereocilium is covered by a tubular sheath of plasma membrane. Although a stereocilium is of constant diameter along most of its length, it tapers over the micrometer or so just above its basal insertion. As the stereocilium narrows from approximately 0.4 μm to one-quarter that diameter, the number of actin filaments diminishes from several hundred to only a few dozen. This thin cluster of microfilaments anchors the stereocilium in the cuticular plate, a thick mesh of interlinked actin filaments lying beneath the cell membrane. Because of this tapered structure, a mechanical force applied at the stereocilium’s tip causes the process to pivot around its basal insertion. Horizontal top connectors, extracellular filaments that interconnect adjacent stereocilia along each of the hair bundle’s hexagonal axes, cause the bundle to move as a unit during stimulation at low frequencies. At high frequencies, the viscosity of the liquid between the stereocilia opposes their separation and again results in unitary motion of the hair bundle.
During its development every hair bundle includes at its tall edge a single true cilium, the kinocilium. This structure possesses at its core an axoneme, or array of nine paired microtubules, and sometimes an additional central pair of microtubules. The kinocilium is not essential for mechanoelectrical transduction, for in mammalian cochlear hair cells it degenerates around the time of birth.
Hair Cells Transform Mechanical Energy into Neural Signals
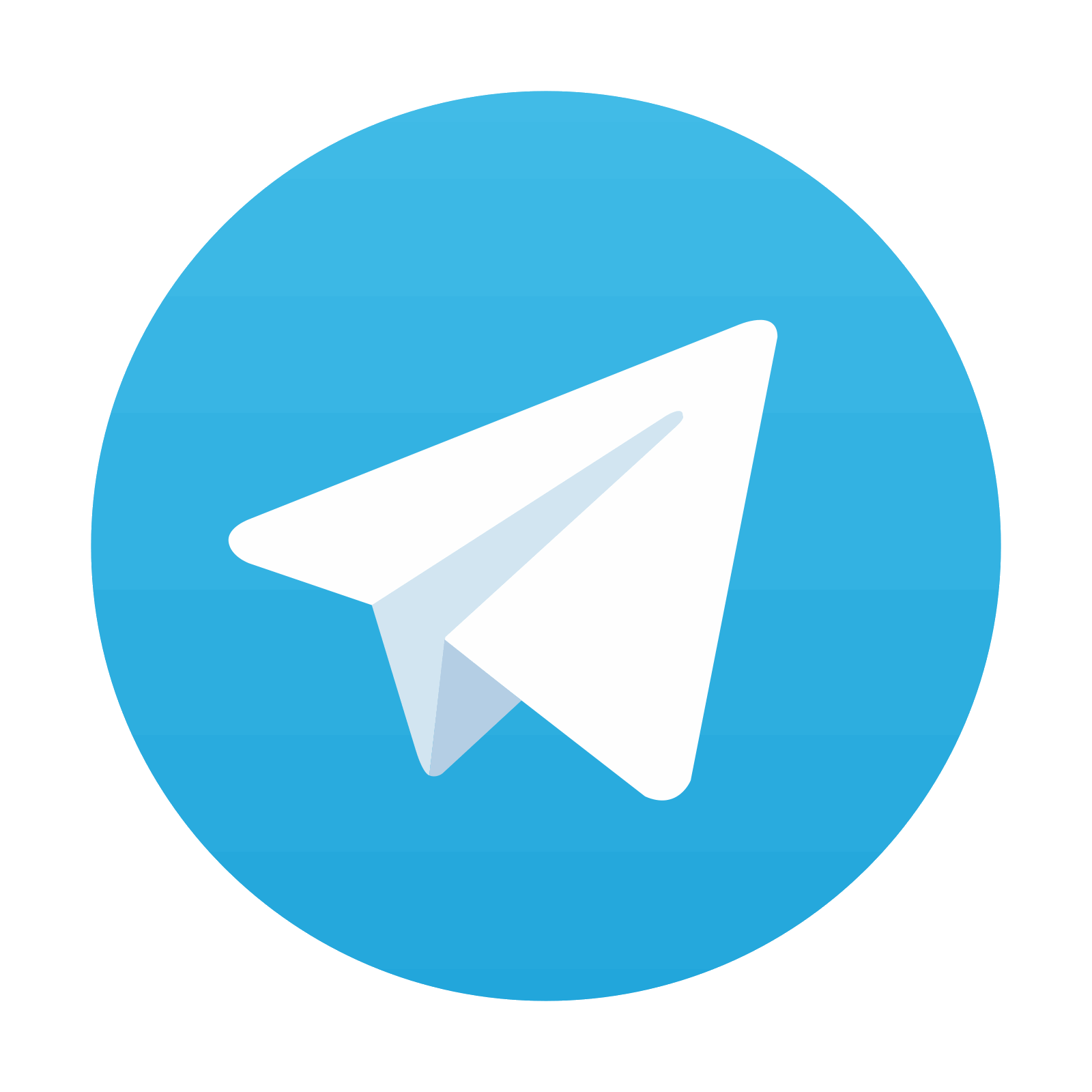
Stay updated, free articles. Join our Telegram channel
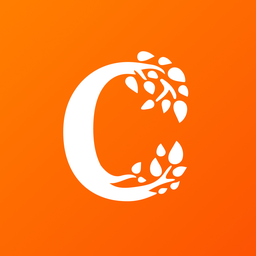
Full access? Get Clinical Tree
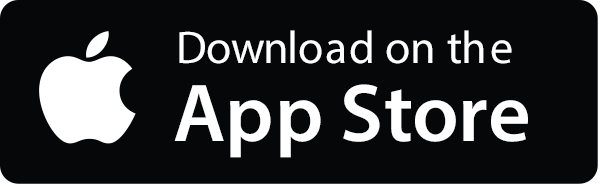
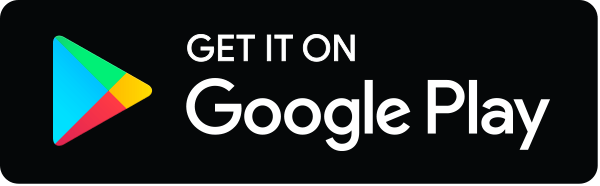