Fig. 1.1
Some key dates and names associated with the history of lithium
Because of the present broad use of lithium in various sectors ranging from high power/energy electrochemical cells, the polymer and glass industries, metallurgy, nuclear activities and medicine (Schou 1957; Birch 1999), this chemical element now stands as essential for our society (Greenwood and Earnshaw 1984). This fully merits applying the powerful tools of modern chemistry and physics to provide a thorough description of its properties both in the metallic state (Li°) and, even more so, of its related ion (Li+).
1.2 The Physico-chemical Properties of Metallic Lithium and Ionized Lithium
1.2.1 Metallic Lithium
As for other chemical elements, most of lithium’s properties can now be qualitatively predicted and understood based on very basic considerations derived from quantum chemistry. These include the electronic structure, size of electronic shells and related monotonous evolution in size, electronegativity, ionization properties and binding properties along rows and columns of the periodic table of the elements. Within this predictive context, the alkali family (group I) is emblematic, as we will now illustrate by describing the fundamental properties of alkali metals, focusing on those of lithium, from which the physical and chemical characteristics of Li+ will be deduced.
At neutral state, the electronic shell of lithium contains only one more electron (Z = 3, 1s22s1) than the nearest inert gas (helium, Z = 2, 1 s2). A similar situation holds for all the elements of the periodic table having this specific [inert gas]ns1 electronic configuration and which form the so-called alkali metal family (Lithium: Li, Sodium: Na, Potassium: K, Rubidium: Rb, Caesium: Cs and Francium: Fr) among which lithium is the lightest. Lithium has the lowest atomic weight of all solids/metals. In the following, the heaviest alkali metal (francium) will be neglected, as none of its known radioactive isotopes has a half-life exceeding 30 min. The size of alkali metals hosting only one outer valence electron (ns1) increases with increasing n, the smaller and larger atomic size being for lithium (1.45 Å) and caesium (2.60 Å), respectively.
The combination of these low atomic weights and large atomic radii explains the very low densities of solid alkali metals all crystallizing in a body cubic-centred (bcc) system under ambient temperature and pressure conditions. Lithium (ρ = 0.53), sodium (ρ = 0.97) and potassium (ρ = 0.86) are the only solid elements less dense than water. Low density and low atomic weight logically impart lithium the highest gravimetric specific heat of all solids (C = 3.6 J∙g−1∙K−1). In its metallic state, the lonely outer s electron bonds weakly with neighbouring atoms, hence (i) the extreme softness of the alkali metals, which can easily be cut with a knife, their hardness ranging from 0.6 (lithium) to 0.2 (caesium) on the Mohs scale, and (ii) the low phase change (melting, vaporization, atomization/sublimation) temperatures and enthalpies (Table 1.1). Based on the increasing atomic size and resulting lower binding energy, these temperatures and enthalpies progressively drop when moving down from lithium to caesium (Table 1.1).
Table 1.1
Some primary selected comparative properties of the alkali elements
Li | Na | K | Rb | Cs | |
---|---|---|---|---|---|
Atomic number (Z) | 3 | 11 | 19 | 37 | 55 |
Fundamental electronic configuration | [He] 2 s1 | [Ne] 3 s1 | [Ar] 4 s1 | [Kr] 5 s1 | [Xe] 6 s1 |
Atomic weight (g∙mole−1) | 6.94 | 22.99 | 39.09 | 85.48 | 132.91 |
Density (solid, 25 °C, g∙cm−3) | 0.53 | 0.97 | 0.86 | 1.53 | 1.90 |
Hardness (Mohs scale) | 0.6 | 0.4 | 0.5 | 0.3 | 0.2 |
Electronegativity (Pauling) | 0.98 | 0.93 | 0.82 | 0.82 | 0.79 |
Melting temperature (°C) | 180 | 97.9 | 63.5 | 39 | 28.4 |
Melting standard enthalpy (kJ∙mole−1) | 3 | 2.6 | 2.3 | 2.2 | 2.1 |
Boiling temperature (°C) | 1336 | 883 | 757.5 | 700 | 670 |
Boiling standard enthalpy (kJ∙mole−1) | 146 | 97 | 80 | 72 | 68 |
Ionization enthalpy eV kJ∙mole−1 | 5.39 | 5.14 | 4.34 | 4.18 | 3.8 |
520 | 496 | 419 | 402 | 376 | |
Standard redox M+/M potential (E°, Volts, 25 °C) | −3.04 | −2.714 | −2.925 | −2.98 | −3.026 |
Flame colour | ![]() |
1.2.2 Ionized Lithium (Li+)
Because of their electronic structure, alkali elements have a clear tendency to lose their sole outer ns1 electron, leading to monovalent M+ ions having a much smaller size, since they reach the electronic structure of the nearest rare gas of the upper (n-1) row (Fig. 1.2). This confers to these monovalent and spherical cations both high stability and poor reactivity. Conversely, alkali metals are highly reactive, unstable and easily oxidized. Their ionization (from neutral M atom to M+ cation) is accompanied by a severe decrease in size. Alkali metals are poorly electronegative (i.e. highly electropositive) as exemplified by potassium (0.82), rubidium (0.82) and caesium (0.79) having the lowest Pauling’s electronegativity of all elements. This intrinsic electronic-driven instability explains why alkali metals can only be naturally found at M+ ionized state, either in salts combined with other elements or dissolved in water. In the absence of any d electron in their electronic shell, alkali ions are noncoloured in aqueous solutions. Moreover, as they do not have unpaired electrons, alkali ions are diamagnetic, but they can be associated with a magnetic anion (e.g. superoxide). In nature, the chemistry of the alkali is mostly that of their monovalent ions. Note that alkali metals are among the few elements, along with fluorine and the alkaline earth elements, which exhibit only one known ionic formal charge. When moving down the alkali metal column in the periodic table, combined shielding and electronic shell size effects weaken the binding of the outermost electron to the nucleus, and the ionization energy (E i) continuously drops accordingly (Table 1.1). The five lowest reported E i among all elements are owned by the alkali metals. Bottom alkali metals (i.e. large n or Z) have E i values reaching the visible light energy range, so that they are used in photoelectric cells or as photosensors in automatic doors, escalators and other electronic devices (caesium). For lithium, ultraviolet light can trigger ionization. Aside from radiation, temperature can also cause ionization, with increasing efficiency when the Z value goes up. It is for this reason that caesium and rubidium can hardly be quantified through atomic absorption spectroscopy, a technique that requires a high level of atomization/excitation with the lowest possible ionization. On the way back to its fundamental ns1 energy level, the ejected electron causes the emission of set of radiations with specific wavelengths, among which the most intense are the 2S1/2 – 2P1/2 and 2S1/2 – 2P3/2 doublet permitted transitions (Moore and Merrill 1968) well known to colour a hot flame. Lithium, sodium and potassium emit carmine/red (λ = 670.7 nm), yellow (λ = 589 nm) and violet/lilac colours, respectively. These specific radiations, which serve as atomic fingerprints, are classically used for qualitative as well as quantitative (flame absorption/emission spectrometry) analysis.
Light and heat trigger the ionization of alkali metals, but this energy conversion is localized and temporary. To be permanent, ionization has to result from the chemical reaction of M with reducible elements/compounds, leading to M+-containing compounds or solutions. This is exemplified by the reaction of M reducing liquid water as follows:
Both the thermodynamics (increase in exothermicity) and kinetics of the reaction of alkali metals with water nicely mirror the drop in E i when travelling down the column: lithium reacts slowly; sodium and potassium set fire; and caesium and rubidium explode. From lithium to caesium, the amount of heat released during this reaction increases, while the metal melting point goes down, so that only lithium does not melt in these conditions. When a flame is spotted, its colour perfectly matches that observed in photometric analysis (Table 1.1).

However, there is an apparent contradiction when comparing the trends in E i and in the M+/M standard redox E° potentials (Table 1.1). For instance, lithium has the highest reducing power based on its E° (−3.04 V vs. SHE), but it has the lowest ability among the alkali metals to lose its outermost 2 s1 electron based on its E i value (5.39 eV). As another example, sodium has the lowest reducing power based on its E° (−2.71 V vs. SHE) while it is slightly less able than lithium to lose its 3 s1 electron (E i = 5.14 eV). Actually, the usual scale of standard redox potential is established in the presence of water; hence, the E° values include the energy of solvation (hydration) of bare M+ ion by the solvent molecules. This contrasts with E i, which only reflects the energy gap between gaseous M(g) and M+ (g). For ease of comprehension, the formation of M+ (aq) from M(s) should be segmented into three steps:
- (i)
The atomization/sublimation of M(s): M(s) → M(g)
- (ii)
The ionization of M(g): M(g) → M+ (g)
- (iii)
The hydration of M+ (g): M+ (g) → M+ (aq)
The variations in free standard energies (ΔG°) associated with each of these steps are well documented in the literature (Latimer et al. 1939; Hush 1948) and show monotonous evolutions as the atomic number (Z) or period number (n) increases, as expected (Fig. 1.3).


Fig. 1.3
Variations in standard free energy (∆G°) for the sublimation (red), ionization (blue), solvation/hydration (green) and sum of the three (black) for each alkali element. Note the resulting out-of-trend behaviour of lithium due to its very exothermic hydration energy
As previously rationalized, ΔG°(i) and ΔG°(ii) decrease as n increases, but the evolution in ΔG°(iii) deserves great attention. It shows a very large negative hydration energy for Li+ (−502 kJ/mol) when compared to ionized sodium (−401 kJ/mol) and then continues to increase more gradually when n or Z increase further. Because of these large values and differences in ΔG°(iii), the sum of the free energies associated with these three steps does not follow a monotonous evolution (Fig. 1.3). Lithium is totally out of scale, because of its exceptional highly exothermic hydration by water, rendering it almost as reducing as caesium. This is shown in Fig. 1.4, which, incidentally, also illustrates the perfect proportionality between the overall total ΔG° (i + ii + iii) and E° (Nernst’s law, ΔG° = – n. F. ΔE°) (Lide 1992). In the absence of any solvation, lithium would be the weakest reducing alkali element.


Fig. 1.4
Correlation between the total free energy for the M(s) → M+ (aq) reaction and the M+/M standard redox potential. The very exothermic hydration reaction of Li+ allows Li to be almost as reducing as Cs
In fact, the large exothermicity of Li+ hydration can be easily accounted for by its very small size (0.6 Å in radius), resulting in its large polarizing power (= charge density = ionic potential) and its strong ability to interact with Lewis-base water (H2O) molecules. The size of the central cavity created by the association of ligands/anions depends on their own size but also on their number/organization: a tetrahedron will delimit a smaller cavity than an octahedron. Li+ is small enough to easily fit in the site at the centre of a tetrahedral association of polar water molecules, with which it will strongly interact. In M(H2O)n + clusters, the size of this cavity (r cavity) hosting the charged M+ ion can be directly computed from the Born equation (Born 1920) bridging r cavity to the free energy of hydration ΔG°(iii) as follows:
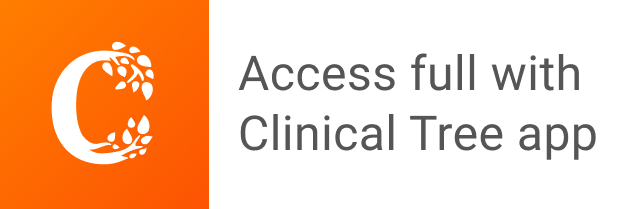