In some studies, the RBC lithium concentration is calculated indirectly using the following equation:
Intra-erythrocytes lithium could be measured directly after RBC separation and haemolysis using one of the previously mentioned methods. Usually, erythrocytes are isolated using density centrifugation and are haemolysed by adding de-ionized water. Proteins are precipitated with trichloroacetic acid and lithium concentrations are measured using FES, AAS or ISE. Internal standards (caesium or potassium) are used routinely to guarantee the quality of FES measurement. However, intra-erythrocyte potassium measurement is preferred by some clinicians to avoid haematocrit variation-related changes. Internal quality controls should cover the infra-therapeutic (0.2–0.4 mmol/L), the therapeutic (0.4–1 mmol/L) and the toxic (≥1.5 mmol/L) zones. Among the commercial quality controls are those of Bio-Rad™, Asqualab™, ProBioQual™, Fumouze™ and Randox™. It is also recommended, and in some countries obligatory, to participate in an external quality control survey.
![$$ \left[\mathrm{R}\mathrm{B}\mathrm{C}\ \mathrm{lithium}\right] = \frac{\begin{array}{l}\ \\ {}\left[\mathrm{whole}\ \mathrm{blood}\ \mathrm{lithium}\right] - \left(\left(1 - \mathrm{hematocrit}\right) \times \left[\mathrm{plasma}\ \mathrm{lithium}\ \mathrm{concentration}\right]\right)\end{array}}{\mathrm{hematocrit}} $$](https://i0.wp.com/neupsykey.com/wp-content/uploads/2017/06/A318968_1_En_2_Chapter_Equb.gif?w=960)
2.3 Peripheral Pharmacokinetics
As described in Chap. 1, lithium is a naturally occurring alkali metal with a small molecular weight (6.941 g/mol) that does not exist in nature as an uncombined free metal form but as a single-charged cation or Li+ associated with counter organic or inorganic ions to form different solid salts. These water-soluble salts give the physicochemical properties of weak bases and hydrophilic characteristics. For the treatment of bipolar disorder, lithium carbonate is the most frequently used lithium salt, but other salts such as acetate, citrate, gluconate and sulphate are also available. Furthermore, lithium carbonate is prescribed in two forms: an immediate-release and a sustained-release preparation. The relative low solubility of lithium carbonate in water, as compared with other salts, has been used to produce sustained-release forms of lithium by decreasing its absorption rate by the intestine. Lithium salts are only available as oral tablets or solution. It is the oral PK properties of lithium salts that are summarized in this chapter, as no intravenous forms of lithium have been available in the market. This chapter reviews the peripheral PK of lithium by considering its absorption, distribution, metabolism and excretion properties (ADME) and then examines several factors that are responsible for lithium PK variability.
2.3.1 Absorption
In clinical practice, lithium is administered by the oral route as tablets or capsules (carbonate) or solution (citrate). Solid pharmaceutical forms of lithium salts include immediate-release and controlled- or extended- or sustained-release forms, depending on the pharmaceutical firm that markets the drugs. Lithium citrate oral solution may be useful in patients unable to swallow capsules or tablets; 5 mL of a commercially available solution contains about 8 mEq of lithium and is approximately equivalent to 300 mg of lithium carbonate. The relatively high water solubility of lithium salts used in therapy permits rapid and total solubility of solid pharmaceutical forms containing lithium salts within the gastrointestinal tract fluids, leading to good oral absorption of lithium – in terms of the amount absorbed by the gut. Moreover, the rate of oral absorption of lithium salts depends on the rate of solubility of the different lithium salts and the pharmaceutical forms, i.e. immediate- or sustained-release forms, within the intestine.
Following oral ingestion, fast and effective absorption is observed within 1–6 h for the immediate-release form (later for the sustained-release form) through the upper gastrointestinal tract (Malhi and Tanious 2011). A complete dissociation of lithium salts occurs after their oral absorption, giving rise to lithium as a single cation in the circulating blood. Chloride and sulphate salts are rapidly and almost completely absorbed, while the less water-soluble carbonate salt is absorbed more slowly (Lehmann 1997). Lithium is mainly absorbed in the jejunum and ileum (Bettinger and Crismon 2006). Although the mechanisms involved are not well known, lithium transport across the intestine involves both active and passive mechanisms (Kersten et al. 1986). The bioavailability of all formulations of lithium is good, with a range of 80–100 % (Hunter 1988; Price and Heninger 1994). Some gastrointestinal factors influence the oral absorption of lithium; for example, food may influence the rate and extent of absorption. Studies by Gai and colleagues (1999, 2000) using two different sustained-release formulations of lithium showed that the extent (bioavailability) of oral absorption was not modified under fasting or fed conditions. However, meals (normal diet, high fat and high fat/protein meals) significantly increased the maximal serum concentration (C max) of lithium as compared with fasting conditions, suggesting that the rate of lithium oral absorption may be increased by meals, even if the observed time of maximum plasma concentration (T max) was similar across fed and fasting conditions (Gai et al. 1999, 2000).
2.3.1.1 Immediate-Release Preparations
After oral administration of a single immediate-release dose of lithium citrate solution or carbonate tablets, T max is observed at approximately 1–3 h and 2–6 h, respectively (Frye et al. 1998; Lee et al. 1998; Thornhill 1978; Ward et al. 1994). Several advantages have been found for citrate solution of lithium over the solid carbonate forms: it increases the rate of lithium absorption, avoids prolonged exposure of the small intestine to lithium and subsequently decreases the gastrointestinal side effects of lithium and decreases interindividual variability of lithium disposition (probably through reduced variability of the absorption of solid forms) (Guelen et al. 1992). Because of their better water solubility, chloride and sulphate in immediate-release solid lithium formulations also peak more quickly than do the immediate-release lithium carbonate forms (Altamura et al. 1977).
2.3.1.2 Sustained-Release Preparations
Sustained-release forms of lithium are prescribed much more frequently in clinical practice than immediate-release forms are, especially in European countries (Grandjean and Aubry 2009a). The first intent of a sustained-release form is to limit high stiff/peak lithium C max that might otherwise reach concentrations in the toxic range and produce a subsequent reduced time in the narrow therapeutic range of lithium concentration (0.6–1.2 mmol/L) (Amdisen 1977; Cooper et al. 1978; Sproule 2002).
After oral administration of a single dose of sustained-release lithium carbonate, T max is extended by between 4 and 12 h as compared with its immediate-release form. The absorption half-life of the immediate-release form is 0.78 ± 0.05 h; for the sustained-release form of lithium carbonate, it is 3.73 ± 0.37 h (Thornhill 1978). Cooper and colleagues (1978) have compared the bioavailability of a slow-release form of lithium with an immediate-release form administered as a single dose or after reaching steady-state conditions in normal volunteers and manic patients. The C max of the slow-release form was delayed (T max = 4.6 ± 0.6 h) compared to the immediate-release form (T max = 1.5 ± 0.2 h). A lowered C max was also observed for the slow-release form (C max = 0.33 ± 0.06 mmol/L) as compared to the immediate-release form (C max = 0.66 ± 0.10 mmol/L). In contrast, lithium absorption, in terms of systemic exposure (area under the curve, AUC), was not statistically significant as the AUC0-48h were similar between the two forms (7.65 ± 1.24 and 6.53 ± 1.38 mmol.h/L for the immediate- and slow-release forms, respectively), indicating equal bioavailability (Cooper et al. 1978).
In a similarly designed study in healthy subjects, the sustained-release preparation was associated with a marked reduction of the C max/C min ratio, which represents fluctuation of lithium serum concentrations at steady state, as comparison with an immediate-release form. But as stated above, lithium serum systemic exposure did not differ between the two forms, with an AUC0-96h of 0.214 ± 0.107 and 0.252 ± 0.097 mmol.h/L for the immediate- and sustained-release forms, respectively (Castrogiovanni 2002). Other studies confirmed the seminal study of Cooper and colleagues showing that, after repeated doses, oral exposure at steady state was similar to that observed after a single administration of an immediate-release form of lithium but with a blunted C max and an increased T max with similar AUC (Hunter 1988; Perry et al. 1981). Importantly, the sustained-release form exhibited higher plasma concentrations than the immediate-release form and reduced interindividual variability by as much as 50 % in bipolar disorder patients (Cooper et al. 1978).
2.3.2 Distribution
Because of their physicochemical properties and small molecular size, lithium ions largely distribute into extracellular and intracellular body water spaces, as do sodium ions. Among all the models tested, the best model to describe lithium serum PK is a two-compartment model with first-order absorption rate constant (ElDesoky et al. 2008; Findling et al. 2010; Sproule et al. 2000; Taright et al. 1994). However, as shown in Table 2.1, some studies have also used a one-compartment model (Hoegberg et al. 2012; Yukawa et al. 1993) or a non-compartmental analysis. However, a lack of robustness of the analytical methods used, and the number of subjects and time points, did not always permit accurate determination of the number of compartments. Lithium, like sodium ion, is water soluble and its binding to plasma proteins is negligible (Clarke et al. 2004; Price and Heninger 1994). Unlike other organic drugs used in mental disorders, lithium has a slow diffusion from extracellular to intracellular compartments due to its low passive diffusion permeability across cell membranes. The means of the central volume of distribution of lithium calculated in several studies were from 0.8 to 1.2 L/kg with an initial distribution into the total extracellular body fluids (Ward et al. 1994). Therefore, lithium has a small volume of distribution, slightly higher than the total body water volume, which makes it susceptible to large variations of total body water volumes that are frequently observed following some pathophysiological changes. For example, with ageing, alteration of body composition such as increased body fat, decreased fat-free mass and total body water will decrease the volume of distribution of lithium, decreasing the time to reach the steady state with repeated doses of lithium together with a reduced elimination half-life and an increase of the C max/C min ratio. Oedemas or, conversely, dehydration syndrome may also change the percentage of total body water and consequently the volume of distribution of lithium. The distribution of lithium into the tissue from the blood occurs variably at differing rates: for example, high and low uptake rates occur in the liver/kidneys and brain/muscles, respectively. The kidney, bone and thyroid lithium concentrations are twice those simultaneously measured in serum (Amdisen 1977; Kusalic and Engelsmann 1999; Wilting et al. 2007) with a T max from 2 to 4 h later than that observed in the serum. Lithium concentrations in the liver and muscles are lower than those in serum and other tissues (kidney, thyroid, bone). Erythrocyte concentrations of lithium correlate in most patients with plasma levels but are subject to large interindividual variability. In early studies, lithium concentration in RBC was proposed as a better surrogate of brain lithium concentration than serum lithium concentration. Today, this statement remains poorly substantiated, and lithium RBC concentrations are not consistently used in clinical practice (Lee et al. 1975; Schreiner et al. 1979; White et al. 1979).
Table 2.1
Main pharmacokinetic parameters of lithium in some studies from the literature in patients with bipolar disorder (mean ± standard error, mean [minimum-maximum] or (% coefficient of variation))
Study | Subject | Salt | PK analysis | Age (years) | BW (kg) | CrCL (L/h) | Dose frequency | Dose (mmol or mg) | C max (mmol/L) | C min, ss (mmol/L) | T max (h) | AUC0-∞ (mmol. min/L) | t 1/2 (h) | CL/F (L/h) | Vd/F (L/kg) |
---|---|---|---|---|---|---|---|---|---|---|---|---|---|---|---|
Single dose | |||||||||||||||
Standard release | |||||||||||||||
Lee et al. (1998) | 8 | Li2CO3 | CA (2C) | 26.9 ± 8.5 | 59.7 ± 11.6 | – | 900 mg | 0.97 ± 0.17 | – | 1.59 ± 0.78 | 722.6 ± 262.7 | 16.3 ± 7.18 | 1.13 ± 0.39 | 1.43 (0.39) | |
Frye et al. (1998) | 13 | – | CA | – | – | – | 600 mg | 0.72 ± 0.15 | – | 1.50 ± 0.91 | 611.4 ± 173.4 | – | – | – | |
Findling et al. (2010) | 39 | Li2CO3 | CA (2C) | 11.9 ± 2.5 | 51.6 ± 16.9 | 7.96 ± 2.67 | 600–900 mg | – | – | – | – | 27.3 (41.0) | 1.34 (51.6) | 39.7a(8.84) | |
At steady state | |||||||||||||||
Standard release | |||||||||||||||
Chapron et al. (1982) | 6 | Li2CO3 | – | 81 [73–88] | 68 ± 14 | 3.74 | Bid | 0.15 mmol/kg/day | – | – | – | – | 28.5 ± 4.9 | 0.83 | z |
Hardy et al. (1987) | 9 | Li2CO3 | – | 73 [67–80] | 56 ± 10 | 4.18 | Od | 0.21 mmol/kg/day | – | – | – | – | 26.9 ± 5.5 | 0.94 | 0.64 (0.16) |
Cooper et al. (1978) | 12 | Li2CO3 | NCA | Adult | Non obese | – | Bid | 24.3–81.0 mmol/day | 0.74 ± 0.15 | ||||||
Sustained release | |||||||||||||||
Cooper et al. (1978) | 12 | Li2CO3 | NCA | Adult | Non obese | – | Bid | 24.3–81.0 mmol/day | – | 0.84 ± 0.17 | |||||
ElDesoky et al. (2008) | 50 | Li2CO3 | CA (2C) | 33 ± 10 | 67 ± 3.6 | 6.3 | Bid | – | – | – | – | – | – | 0.51 | 15.2a |
Hoegberg et al. (2012) | 11 | Li3C6H5O7 | CA (1C) | 52 [21–80] | 72 [45–125] | Bid | Adjusted dose | 0.74 (0.19) | |||||||
Potkin et al. (2002) | 10 | Li2CO3 | CA | 32.8 ± 1.9 | 87.6 ± 3.3 | – | Bid | 16.2–64.8/day | 1.11 | 0.55 [0.32–0.77] | 1.07 [0.55–4.07] | 554 [408–750]/12 h | – | – | – |
2.3.3 Metabolism
Lithium is not subject to first-pass hepatic metabolism (Bauer et al. 2006). A small amount (2 %) of lithium is eliminated in the bile within 24 h of its oral administration (Terhaag et al. 1978). Thus, the PK of lithium is not influenced by any changes in hepatic biotransformation or hepatic blood flow.
2.3.4 Excretion
Lithium is freely filtered by the renal glomerulus with a filtration rate similar to that of other small molecules circulating freely in the plasma that are unbound to proteins. Once filtered, about 75–80 % of lithium is actively reabsorbed in the proximal tubules, while a small proportion can also be reabsorbed in loop of Henle and by the epithelial channel which involves sodium (eNaC) in the early distal tubules (Hayslett and Kashgarian 1979) under sodium-restricted conditions (Thomsen and Shirley 2006; Trepiccione and Christensen 2010). The rate of lithium renal elimination is linearly correlated with its serum levels (Bauer et al. 2006). The terminal elimination half-life (t 1/2) of lithium in the plasma ranges from 16 to 30 h in healthy volunteers and patients with normal renal function (Arancibia et al. 1986; Cooper et al. 1978; Granneman et al. 1996; Hunter 1988; Lee et al. 1998; Luisier et al. 1987; Thornhill 1978). This elimination phase can be observed as early as 4–6 h after the T max. The lithium elimination half-life during long-term lithium treatment or in patients with impaired renal function increases and can reach 50 h. A linear relationship between the dose and the AUC of serum lithium levels versus time has been determined following several oral doses of lithium (Amdisen 1977); this linear relationship is an indicator of any saturation of the different PK phases (absorption, distribution, elimination) of lithium at usual therapeutic doses (12–36 mmol/24 h). However, this does not argue against the involvement of carrier-mediated processes in absorption, distribution and excretion, as sufficient concentrations to saturate and kinetically reveal these transport systems could not easily be reached in vivo.
Lithium is mainly eliminated by the kidneys as a free ion. Its clearance ranges from 0.6 to 2.4 L/h (Ward et al. 1994) with large interindividual variability (Amdisen 1977; Frye et al. 1998; Perry et al. 1981). This variability has been identified in PK population studies (Taright et al. 1994; Yukawa et al. 1993), interindividual lithium clearance variability being from 25 % to 38 % in bipolar patients with normal renal function. Lithium total body clearance has been reported as variable depending on several pathophysiological states, such as age, total body weight and renal function (ElDesoky et al. 2008). It has been clearly demonstrated that the effect of weight parameters (total body weight or lean body weight) and creatinine renal clearance accurately predict steady-state lithium concentrations by decreasing interindividual variability (Sproule et al. 2000). Renal lithium clearance ranges from 10 to 40 mL/min and decreases with age (linearly with age-related decrease in renal glomerular filtration), low sodium intake, volume depletion in dehydration or with age, cardiac failure, concomitant use of drugs affecting renal glomerular filtration such as nonsteroidal anti-inflammatory agents (see Sect. 2.5.1.3), kidney disease and any event increasing proximal tubular reabsorption of sodium and water (i.e. dehydration, decreased salt intake, extra-renal salt loss, diuretics) and increased with pregnancy and total body weight.
2.4 Factors Influencing the Pharmacokinetics of Lithium
Intra-subject variability in lithium PK is low in patients who receive lithium and achieve constant normal renal clearance during administration of the medication. However, several physiological and co-medication factors can contribute to high interindividual variability in lithium PK. This latter variability necessitates individualization of lithium dosage regimens and close monitoring of lithium serum concentrations to avoid no response or, conversely, severe adverse effects of lithium therapy. This section summarizes the major factors that influence lithium PK.
2.4.1 Renal Function
In the case of significant impaired renal function, plasma lithium concentrations increase quickly and may trigger acute toxicity symptoms. A preliminary check of renal function is thus highly recommended before initiation of lithium treatment, followed by regular checks throughout the course of treatment (see Sect. 2.8). Lithium by itself causes a modest decline in renal function, thus increasing its elimination half-life and steady-state levels. End-stage renal disease (ESRD) is a very rare complication of long-term lithium treatment affecting only 1 % of patients over 15 years of treatment (Tredget et al. 2010). No study has evaluated the variability of the PK parameters for patients with ESRD. A known renal failure after lithium initiation is considered a contraindication, especially when a sodium-poor diet is also prescribed (Grandjean and Aubry 2009a).
2.4.2 Body Weight
Reiss and colleagues (1994) found that, in obese patients, the steady-state volume of distribution in litres was not significantly different from that in normal weight individuals (44.5 ± 9.8 L versus 41.3 ± 8.4 L in obese and nonobese subjects, respectively), which is consistent with the similarly calculated ideal body weight (IBW), and thus significantly less as expressed in litres/kg (0.42 ± 0.09 L/kg and 0.66 ± 0.16 L/kg for obese and nonobese patients, respectively). Total lithium clearance was greater in obese patients (33.9 ± 7.0 mL/min in obese patients versus 23.0 ± 6.2 mL/min in nonobese subjects). The authors thus demonstrated that the volume of distribution of lithium was significantly correlated with ideal body weight and fat-free mass but not with total body weight. Lithium clearance was significantly correlated with total body weight but not with creatinine clearance. The major conclusion was the necessity to increase the maintenance dose in obese patients to achieve similar lithium steady-state levels as those observed in nonobese patients, because of a higher lithium clearance in obese patients. However, the starting dose should be the same as that used in nonobese patients since the volume of distribution of lithium in obese patients is more related to the ideal body weight than the total body weight.
2.4.3 Circadian Rhythm
Circadian rhythm has a specific influence on the PK of lithium. A study in rats by Olesen and Thomsen (1985) reported a significant decrease in serum lithium concentration during the early night portion of the light-dark cycle, when rats are most active, associated with a large increase in renal clearance of lithium. In mice, the toxicity of acute intraperitoneal injection of lithium chloride (LiC1) (940 mg/kg) increased from 20 % during the night to 70 % during the day (Hawkins et al. 1978). Similarly, Shito and colleagues (1992) reported time-dependent changes in both lithium clearance and toxicity. Chapter 6 is devoted entirely to this topic.
2.4.4 Age
Elderly bipolar disorder patients require a lower dose of lithium to achieve the same steady-state levels observed in young adults with normal renal clearance (see Sect. 2.6.2). In contrast, child bipolar disorder patients have a shorter elimination half-life and greater clearance compared to adults (see Sect. 2.7.1).
2.4.5 Cardiovascular Disease
Associated with age, hypertension and congestive heart failure may decrease the glomerular filtration rate and accordingly further reduce lithium renal excretion (Sproule et al. 2000), indicating that a lower dose of lithium may be required in these patients.
2.4.6 Pregnancy and Lactation
Briefly, lithium clearance increases by 30–50 % in pregnant women due to an increase in its renal glomerular filtration rate, especially during the last months of pregnancy. There is a rapid return to the pre-pregnancy state immediately after delivery (Grandjean and Aubry 2009b; Schou 1990; Viguera et al. 2002). Therefore, TDM must be carried out more frequently for pregnant women. Lithium also diffuses freely from maternal plasma to breast milk (Schou 1990; Yoshida et al. 1999) with serum concentrations in infants ranging from 10 % to 50 % (and possibly up to 80 %) of those in maternal plasma (Viguera et al. 2002). All studies in pregnancy and breastfeeding populations are necessarily retrospective, with the safety of the infant-mother couple being the first concern. (See Chap. 18.)
2.5 Drug-Drug Interactions (DDIs)
Drug-drug interactions involving lithium arise from pharmacodynamic and/or PK interactions. In this section, we will focus on DDI of PK origin that affect lithium serum concentrations. As lithium is absorbed well by the gastrointestinal tract, not metabolized, not bound to plasma proteins and almost entirely eliminated from the body through renal excretion, the major DDIs of PK origin are due to modifications of lithium renal glomerular filtration and/or tubular reabsorption. Competition between sodium and lithium for their tubular reabsorption processes is also a critical key element for lithium renal excretion, and drug-induced sodium depletion is one of the main mechanisms modifying lithium renal clearance.
2.5.1 Drugs That Decrease Lithium Clearance
2.5.1.1 Thiazide and Thiazide-Like Diuretics
Thiazide and thiazide-like diuretics (chlorothiazide, hydrochlorothiazide, bendroflumethiazide, chlorthalidone, indapamide, methyclothiazide, metolazone, polythiazide) are inhibitors of sodium reabsorption in the distal tubules. The mechanism by which lithium serum concentrations are potentially increased with co-medication of these diuretics is directly linked to a compensatory up-regulation of sodium (and thus lithium) reabsorption in the proximal tubules. This has been clearly demonstrated for chlorothiazide, which reduces the renal clearance of lithium by 40–70 %, depending on the diuretic doses. Co-medication of lithium (300 mg of lithium carbonate) alone and with chlorothiazide (500 mg/day for 1 week) was studied in healthy volunteers. Chlorothiazide increased lithium plasma concentration by 26.2 % and decreased renal lithium clearance by 26.5 % (Poust et al. 1976). Subsequently, it was reported in healthy volunteers (Crabtree et al. 1991) that hydrochlorothiazide (25 mg twice daily) in combination with lithium for 5 days increased average lithium serum concentrations by 23 %. Therefore, DDI of lithium with thiazide diuretics has been extended to other thiazide and thiazide-like diuretics even in the absence of ad hoc clinical studies. As a general rule, clinicians reduce (usually halve) the dose of lithium once a thiazide diuretic is added to the medication regimen, and serum lithium concentrations should therefore be more closely monitored. Another clinical approach may simply be to avoid these diuretics in patients with cardiac disorders, as so many alternatives are now available on the market for this indication.
2.5.1.2 Inhibitors of Angiotensin-Converting Enzyme (ACE) and Angiotensin-Receptor Antagonists
Inhibitors of ACE are drugs widely used in the treatment of hypertension and heart failure and as protective agents against renal failure diabetes mellitus. The effect of ACE inhibitors on lithium renal clearance is variable and reports on toxicity have been less common than with thiazide diuretics. However, in the early 1980s, several case reports evidenced an increase in lithium serum concentrations together with lithium toxicity once co-medication with ACE inhibitors (enalapril, captopril, lisinopril) was initiated in the following days or weeks. This has been reviewed thoroughly by Lehmann and Eberhard, while the molecular mechanism of the DDI remains poorly understood (Lehmann and Ritz 1995). A decrease in aldosterone plasma levels observed in patients treated with lithium may induce sodium depletion and thus lithium retention. DDI between enalapril and lithium has been studied in the only prospective study analysing the effect of enalapril for 10 days on lithium serum concentrations in nine healthy volunteers. However, only one patient exhibited a 31 % increase in lithium serum concentrations when enalapril was added, whereas there was no statistical difference for the other eight patients (DasGupta et al. 1992). Another longitudinal case-control study of 20 hypertensive patients previously stabilized on lithium therapy evaluated the effect of ACE inhibitors (lisinopril, enalapril and captopril) on steady-state lithium concentrations and lithium toxicity signs. After initiation of the ACE inhibitor, steady-state lithium concentrations increased by 36.1 %, and four patients presented symptoms suggestive of lithium toxicity (Finley et al. 1996). The clinical significance of this DDI between lithium and ACE has been reported in a population-based study that included 413 patients admitted to hospital at least once for lithium toxicity. A dramatically increased risk of lithium toxicity was observed within a month of introducing an ACE inhibitor (Juurlink et al. 2004). Several case reports have also described potential DDI between angiotensin-2 receptor antagonists (losartan, valsartan, telmisartan).
2.5.1.3 Nonsteroidal Anti-inflammatory Drugs (NSAIDs)
As for ACE inhibitors, the mechanism of action of this DDI remains poorly understood. The possible main mechanism by which NSAIDs increase serum lithium concentrations is directly linked to the inhibition of cyclooxygenase (COX) in the kidney reducing the plasma levels of prostaglandin E2 (PGE2). Reduction in PGE2 levels by NSAIDs decreases the renal blood flow and subsequently the glomerular filtration rates of lithium; it may concomitantly mediate sodium retention as well as that of lithium. Furthermore, micropuncture experiments showed that dosing rats with indomethacin increased the reabsorption of lithium in loop of Henle; this latter effect might be another mechanism by which NSAIDs lower lithium renal excretion (Boer et al. 1993). The effect of NSAIDs on lithium clearance was reported for the first time at the end of the 1970s, where indomethacin increased plasma lithium concentrations by 59 % in three psychiatric patients and by 30 % in four volunteers (Frölich et al. 1979). Diclofenac produced a similar effect, decreasing lithium renal clearance by 23 % and increasing lithium plasma levels by 26 % in women treated with lithium (Reimann and Frölich 1981) – in contrast to aspirin and sulindac, which had no effect (Ragheb and Powell 1986; Reimann et al. 1983). Other NSAIDs have also been investigated for their effect on serum lithium concentrations. Several case reports and clinical studies have reported that naproxen (Ragheb and Powell 1986), piroxicam (Kerry et al. 1983) and selective inhibitors of COX-2 such as celecoxib (Slørdal et al. 2003) and rofecoxib (now withdrawn from the market) (Lundmark et al. 2002) increased serum lithium concentrations.
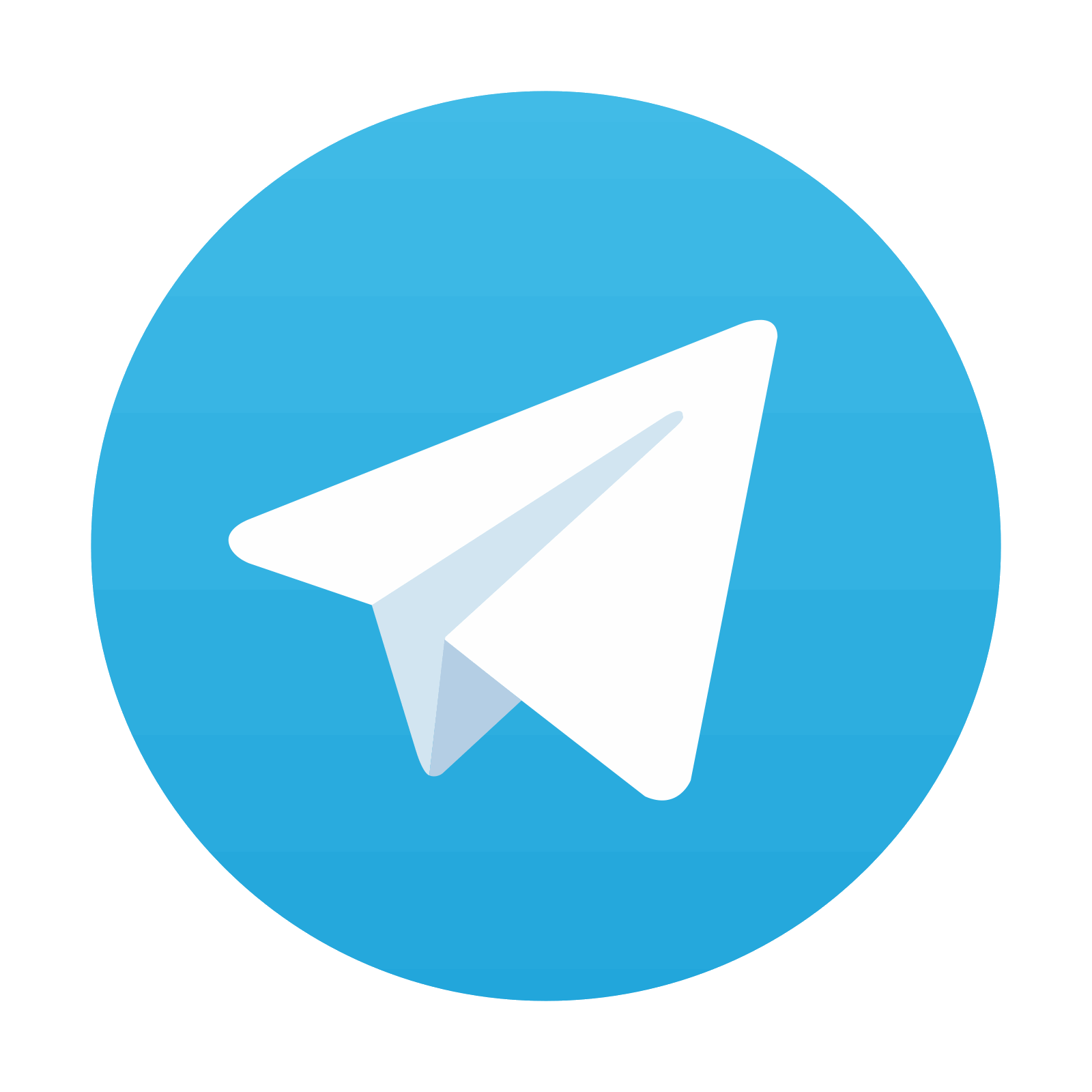
Stay updated, free articles. Join our Telegram channel
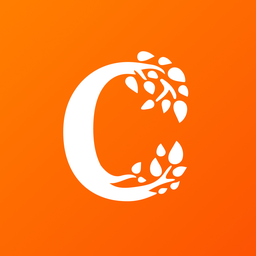
Full access? Get Clinical Tree
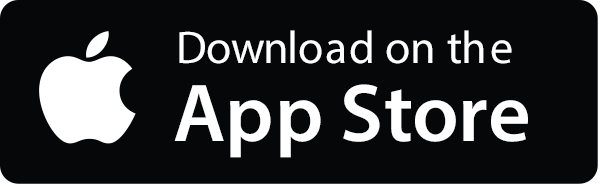
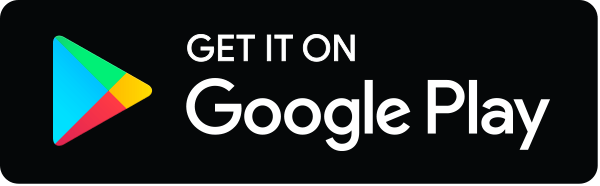