The Study of the Brain
Your brain is a machine whose complexity far exceeds anything constructed by human hands. It is made up of units – cells called neurons – that provide both the pathways by which information is transmitted within the brain and the computing machinery that makes it work. There are quite a lot of these neurons: about 30 times as many as the entire population of this planet. Another not very useful fact to impress your friends with is that every cubic inch of your cerebral cortex has 10 000 miles of nerve fibre in it. A typical neuron is wired up to a thousand or two of its neighbours, and it is the pattern of these connections that determines what the brain does. All this makes the brain a fantastically complex structure, a thicket of twisting, interweaving fibres.
Our brains need to be complex: part of what they do is to embody a working model of the outside world, that enables us to imagine in advance what will be the result of different courses of action. It follows that the brain must be at least as complicated as the world we experience. So the study of the brain is like the study of human society: for a society can only be fully understood if we comprehend not just the behaviour of isolated individuals, but also the interactions that each of them makes with others. Understanding the brain is a task as daunting as trying to comprehend the behaviour of the entire human race, its politics, its economics and all other aspects of what it does; in fact, about 30 times more difficult. As a result, the study of the brain has in some respects a closer affinity with ‘arts’ subjects such as history than it does with much conventional science. For many people that is its attraction. 

The need for neurons
How has such complexity come about? The evolutionary history of the brain is not well understood, but its broad outline was certainly something like the following.
For a single-celled organism such as an amoeba, coordination is essentially chemical: its brain is its nucleus, acting in conjunction with its other organelles.
But a multicellular organism clearly needs some system of communication between its cells, particularly when, as in the primitive invertebrate Hydra, they are specialized into different functions: secretion, movement, nutrition, defence and so on. Communication between cells practically always means chemical communication. One cell releases a chemical substance; somewhere else another cell is waiting for this chemical, which tells it to do something. Nearly always, the target cell has receptor proteins in its cell membrane that recognize the particular chemical. Often these form part of what are technically called ligand-gated channels in the membrane, opening in response to the chemical and often allowing ions such as calcium to enter the cell, which act as messengers. This kind of chemical communication is most familiar as hormones, operating within the body, perhaps to cause widespread changes: a good example is adrenaline, released in dramatic circumstances and alerting more or less the whole body. The chemical is not directed at any particular cell: it is broadcast throughout the whole body.

Diffusion time
In small and slow creatures, this works fine. The one shown below has sensory cells (S) that control motor cells (M) by releasing a chemical transmitter or hormone into the common fluid space. But as an organism gets bigger, things get difficult, in two ways. First of all, time: how long it takes for the message to get from the sender cell to its recipient depends on diffusion. Depends rather
dramatically, because diffusion time is proportional to the square of the distance travelled – Einstein’s law – so it takes a disproportionately longer time for a chemical signal to travel over increasing distances: 1 µm takes 0.5 ms, but 10 mm takes 15 hours. If speed of response is not particularly important, this kind of communication may still be satisfactory even in very large organisms: our own hormonal control systems are of course precisely of this kind. Circulation helps, of course, but even so your blood takes about a minute to go once round the body. If a tiger suddenly burst into your room, although you would instantly release a burst of adrenaline into your bloodstream, it would take about a minute for it to reach its target tissues. By that time you might well have been devoured.
dramatically, because diffusion time is proportional to the square of the distance travelled – Einstein’s law – so it takes a disproportionately longer time for a chemical signal to travel over increasing distances: 1 µm takes 0.5 ms, but 10 mm takes 15 hours. If speed of response is not particularly important, this kind of communication may still be satisfactory even in very large organisms: our own hormonal control systems are of course precisely of this kind. Circulation helps, of course, but even so your blood takes about a minute to go once round the body. If a tiger suddenly burst into your room, although you would instantly release a burst of adrenaline into your bloodstream, it would take about a minute for it to reach its target tissues. By that time you might well have been devoured.
![]() |
![]() |
Specificity
The other problem is specificity: that little creature can do just two things, but we can do much more. Each of us has well over 1200 separate muscles, for a start. And as we get more complicated, with lots of different receptor cells and lots of different muscle cells, we want to do more specific things in response to specific circumstances. In a hormonal system, specificity can only come about by having a wide range of different chemicals, with targets cells responsive to some but not others. In fact the list of hormones in our bodies is already a very long one, running into hundreds: but still not enough for the countless different actions we might want to undertake. What is the solution?
In hormonal systems, a message is in effect shouted throughout the whole body. One way of being more specific is to whisper it confidentially into the ear of the target cell. And this is where neurons come in. Neurons are simply cells with special elongated output processes called axons, which can often be very long indeed – the longest neurons in your body measure about a metre long. The axons grope out towards their targets and make physical contact with them at regions called synapses. They still release their chemical at the synapse, but this time it only affects the cells it makes contact with and not the others: so we no longer need lots and lots of different transmitters. For instance, every muscle in your body is controlled by the same chemical substance, acetyl choline. Though specific, neurons can still be widespread in their actions: because axons can branch, they are capable of influencing thousands of target cells that can be scattered over a very wide area. These axons end either on muscles (where the synapse is called a neuromuscular junction), on the cell body (soma) of a target neuron, or often on the tree-like dendrites that enormously increase the surface area of many neurons.
![]() |
![]() |
It is still chemical communication: but we call the chemicals transmitters rather than hormones. There are several dozen known transmitter substances, falling into three main groups: amino acids, amines and peptides. In fact, knowing the name of a transmitter doesn’t tell you much, because transmitters don’t have to do the same thing at every site. The receptor molecules on the target cell translate arrival of the transmitter into some specific action, but different cells may use different receptor proteins and therefore translate the event differently. Thus there is no necessary logical connection between what the transmitter is and what it does. For example, acetyl choline excites skeletal muscles but inhibits heart muscle: the receptor proteins in the membrane of heart muscle cells are different from those in skeletal muscle. The message is the same, but the meaning is different.
So nerves provide a system that is very specific both in what it does and where it does it. But what about speed? As we have seen, diffusion down long neurons would be hopelessly slow for distances of more than a few microns. Yet the fastest neurons can actually convey information
at over 100 m/s. They are able to do this because they use a much quicker physical process than diffusion, namely electricity and the flow of current: these processes are discussed in the next chapter.
at over 100 m/s. They are able to do this because they use a much quicker physical process than diffusion, namely electricity and the flow of current: these processes are discussed in the next chapter.
Clinical box 1.1 Neurotransmitters as drug targets
The issue of specificity is one of the first hurdles facing the clinician. As noted above, there are only a limited number of transmitter chemicals to bear a near infinite number of bits of information. The evolutionary solution has been the neuron, giving precision to the signal using spatial coding by delivering the transmitter to a precise recipient. Rather as the order ‘fire’ is one of a limited number of commands a sniper might expect to hear in the field, the general accomplishes specificity by ensuring the command is issued to the correct person; confusing his or her cables could clearly deliver a suboptimal result. The problem the clinician faces when trying to restore brain function by using administered analogues of neurotransmitters is that of targeting the signal to the correct recipient.
For example, morphine acts on a family of receptors, and mimics endogenously generated signals called opioids. We know morphine as a rather powerful painkiller, and, not surprisingly, it acts on neurons that block pain signalling. Unfortunately, there are other morphine receptors located elsewhere in the body, the activation of which has rather less desirable characteristics; for example, neurons exist in ganglia near the bowel which, when activated by morphine, reduce gut motility. Indeed, one of the most distressing side-effects of morphine is constipation. The body gets around this problem by targeting its opioid release to those sites where it is needed – pain pathways when pain is disabling, bowel ganglia when gut activity is excessive. The clinician does not have the option of targeting individual neurons in this way and most side-effects of neurological drugs stem from this lack of specificity (see treatment of Parkinson’s disease and the use of benzodiazepines discussed in Chapter 3, p. 51).
The brain
The basic ground-plan of the brain
In vertebrates, neurons develop from the ectoderm, the outer layer of embryonic germ cells that also forms skin. Some remain in epithelia as sensory receptors that are sensitive to mechanical or chemical stimuli, to temperature or to electromagnetic radiation. Others migrate inwards, and become specialized as interneurons, that respond only to the chemicals released locally by sensory neurons or other interneurons. They in their turn release transmitter at their terminals, which form synapses either with interneurons or with effectors such as muscles or secretory cells. So interneurons provide the communication channels by which information is passed rapidly from one part of the central nervous system to another, through mechanisms that will be discussed in Chapter 2 and Chapter 3. In Hydra, for example, we find a network of such intercommunicating neurons, making contact on the one hand with sensory cells on its surface that respond to touch and chemical stimuli, and on the other with muscle cells and secretory glands. Hydra‘s brain is thus spread more-or-less uniformly throughout its body, with only a slight increase in density in the region of its mouth: yet even such a relatively undifferentiated structure can generate well-coordinated, even ‘purposeful’, behaviour.
The next step in the evolution of the nervous system came with the increasing specialization of sensory organs, particularly of teloreceptors such as eyes and olfactory receptors. For an animal that normally moves in one particular direction, as in the earthworm above, such organs tend to develop at the front end, and the result of the consequent extra influx of sensory information to a localized region is an increased proliferation of interneurons in the head. In Planaria we see the first true brain of this kind, a dense concentration of neurons close to the eyes and sensory lobes of the head, giving rise to a pair of nerve cords that run down the body and send off side branches connecting with other neurons and effector cells. In segmented animals like the earthworm, the nerve cords show a series of swellings or ganglia, one to each segment. Each is a kind of brain in its own right, and a decapitated earthworm is still capable of many kinds of segmental and intersegmental coordination. Though our own bodies are not of course segmented, our nerve cord, the spinal cord, still shows some segmental properties, particularly in the organization of the incoming and outgoing fibres, and in the existence of corresponding chains of ganglia along each side. We shall see later, in Chapter 10, that our spinal cord is also capable of a limited degree of brain-like activity. The primitive nerve net has
not been altogether superseded, but survives as an adjunct to the central nervous system in the diffuse networks near the viscera that control movements of the gut and some other visceral functions (for example, stretching the gut causing contractions) and in the ancient diffuse core of the brain, the reticular formation.
not been altogether superseded, but survives as an adjunct to the central nervous system in the diffuse networks near the viscera that control movements of the gut and some other visceral functions (for example, stretching the gut causing contractions) and in the ancient diffuse core of the brain, the reticular formation.
![]() |
The subsequent development of the brain is rather more complex, and not well understood. By looking at its evolutionary history in conjunction with the sequence of its growth in fetal development, one can postulate a framework that may help to relate the primitive nervous system to the more intricate structure of the adult human brain. 

![]() |
The central nervous system is derived from a narrow strip of ectoderm, the neural plate, that runs down the middle of the vertebrate embryo’s back (a, above). The centre of this strip becomes depressed into a trough or groove, and eventually its edges come to meet in the middle to form a closed structure, the neural tube (b; sensory regions shown green, motor regions, blue). Quite a common defect, spina bifida, occurs as a result of failure of this process of closure in the spinal cord; higher up, a similar defect produces, fatally, anencephaly. It is natural for sensory fibres from the skin to enter at the margins of the neural plate, and for motor fibres to the more medial musculature to leave the plate nearer the midline, and as a consequence one finds that it is in the dorsal half of the neural tube that the sensory fibres terminate (their cell bodies lying in the dorsal root ganglia on each side of the tube, c), while the cell bodies of the efferent motor fibres lie in the ventrolateral part of the neural tube: in between are afferents and efferents to the viscera. This dorsoventral arrangement can be traced right up to the highest levels of the brain, but is particularly evident in the adult human spinal cord. Here one can see in cross-section (at the level of the second thoracic vertebra) the ventral and dorsal horns, consisting of masses of grey matter (mostly cell bodies), a less prominent central region concerned with the neural control of visceral function, and a surrounding sheath consisting of white matter, mainly bundles of nerve fibres running longitudinally up and down the cord. If one visualizes a highly schematized hypothetical creature like the rather cute one below, the topological reasons for these structural relationships become obvious.
![]() |
![]() |
At the head end, the process of cephalization mentioned earlier causes the basic plan of the neural tube to be modified. The central fluid-filled canal, which is very small in the spinal cord, widens out at two separate points to form hollow chambers or ventricles: at the same time it migrates back to the dorsal surface of the neural tube, so that the ventricles are open on their dorsal side. As a result, the motor areas are most
lateral, the sensory areas most medial, and visceral areas in between. The surface of the ventricle is covered by the choroid membrane, the site of production of the cerebrospinal fluid that fills the canals and ventricles of the brain. The more caudal of the ventricles is called the fourth ventricle, and the region around it is the hindbrain or rhombencephalon; it is connected to the more rostral third ventricle by the cerebral aqueduct. The region round the aqueduct is called the midbrain or mesencephalon, and that round the third ventricle is the forebrain or prosencephalon. The hindbrain is likewise divided into two regions: the caudal part is called the medulla, and the rostral part of it (metencephalon) is marked by the out growth of the cerebellum over the dorsal surface, and a massive bundle of fibres associated with it, the pons, on the ventral surface. Subsequently, the third ventricle produces a pair of swellings at the front end, which become inflated into the lateral ventri cles: the neural tissue surrounding them forms the cerebral hemispheres (telencephalon) while the rest of the forebrain is called the diencephalon.
lateral, the sensory areas most medial, and visceral areas in between. The surface of the ventricle is covered by the choroid membrane, the site of production of the cerebrospinal fluid that fills the canals and ventricles of the brain. The more caudal of the ventricles is called the fourth ventricle, and the region around it is the hindbrain or rhombencephalon; it is connected to the more rostral third ventricle by the cerebral aqueduct. The region round the aqueduct is called the midbrain or mesencephalon, and that round the third ventricle is the forebrain or prosencephalon. The hindbrain is likewise divided into two regions: the caudal part is called the medulla, and the rostral part of it (metencephalon) is marked by the out growth of the cerebellum over the dorsal surface, and a massive bundle of fibres associated with it, the pons, on the ventral surface. Subsequently, the third ventricle produces a pair of swellings at the front end, which become inflated into the lateral ventri cles: the neural tissue surrounding them forms the cerebral hemispheres (telencephalon) while the rest of the forebrain is called the diencephalon.
![]() |
Table 1.1 Some important structures within the three divisions of the primitive brain | ||||||||||||||||||||||||||||||||||||||||||||||||
---|---|---|---|---|---|---|---|---|---|---|---|---|---|---|---|---|---|---|---|---|---|---|---|---|---|---|---|---|---|---|---|---|---|---|---|---|---|---|---|---|---|---|---|---|---|---|---|---|
|
![]() |
In less-developed species such as fish all these structures are easily recognizable without dissection, but in humans the extraordinary ballooning growth of the cerebral hemispheres has not only engulfed the other surface features (leaving only the cerebellum and medulla peeping out at the back), but the massive fibre tracts needed to connect the cerebral hemispheres to each other and to the rest of the brain have tended to elbow older structures out of the way and have often considerably distorted their shape. Another factor that makes the anatomy of the human brain somewhat confusing is that the neural tube has become bent forward: while the axis of the hindbrain is near vertical, that of the forebrain is horizontal.
An overview of the brain’s structure
The most important areas of the human brain are shown in the sections below: sagittal (showing the medial aspect), and transverse (in the plane of the dotted pink line).
Let us have a preliminary look at what the main bits actually do.


![]() |
![]() |
The cerebellum is an important coordinating system for posture and for motor movements in general (Chapter 12), which arose originally as an adjunct to the vestibular apparatus, a sensory organ concerned with balance and the detection of movement (Chapter 5). Important landmarks on the dorsal surface of the midbrain are the four bumps (corpora quadrigemina) formed by the superior and inferior colliculi (‘colliculus’ = ‘little hill’), primitive sensory integrating areas for vision and hearing respectively; in higher vertebrates their function is mainly that of organizing orienting reactions and other semireflex responses to visual and auditory stimuli. In the diencephalon, on each side of the third ventricle, lie the two halves of the thalamus (‘inner chamber’), a dense group of nuclei whose neurons partly act as relays for fibres that project upward to the cerebral hemispheres. Close to them but more lateral is the corpus striatum, an old area that is concerned with the control of movements (Chapter 12). Also in the diencephalon, but lying on the floor of the third ventricle, is the hypotha lamus, the brain’s interface with the hormonal and autonomic systems that control the body’s internal homeostasis (Chapter 14). More laterally, various nuclei, fibre tracts and other areas form a loosely defined system called the limbic system, which connects both with the hypothalamus, with the olfactory areas, and with many other regions of the brain; they are concerned with such functions as emotion, motivation and certain kinds of memory (Chapter 14). Finally, there is the cerebral cortex (‘cortex’ = ‘rind’), that covers the lateral ventricles and is deeply convoluted and furrowed in higher vertebrates, enabling a large superficial area of tissue to be crammed into a relatively small volume. Its role in carrying out some of the most complex things we do is discussed in Chapter 13.
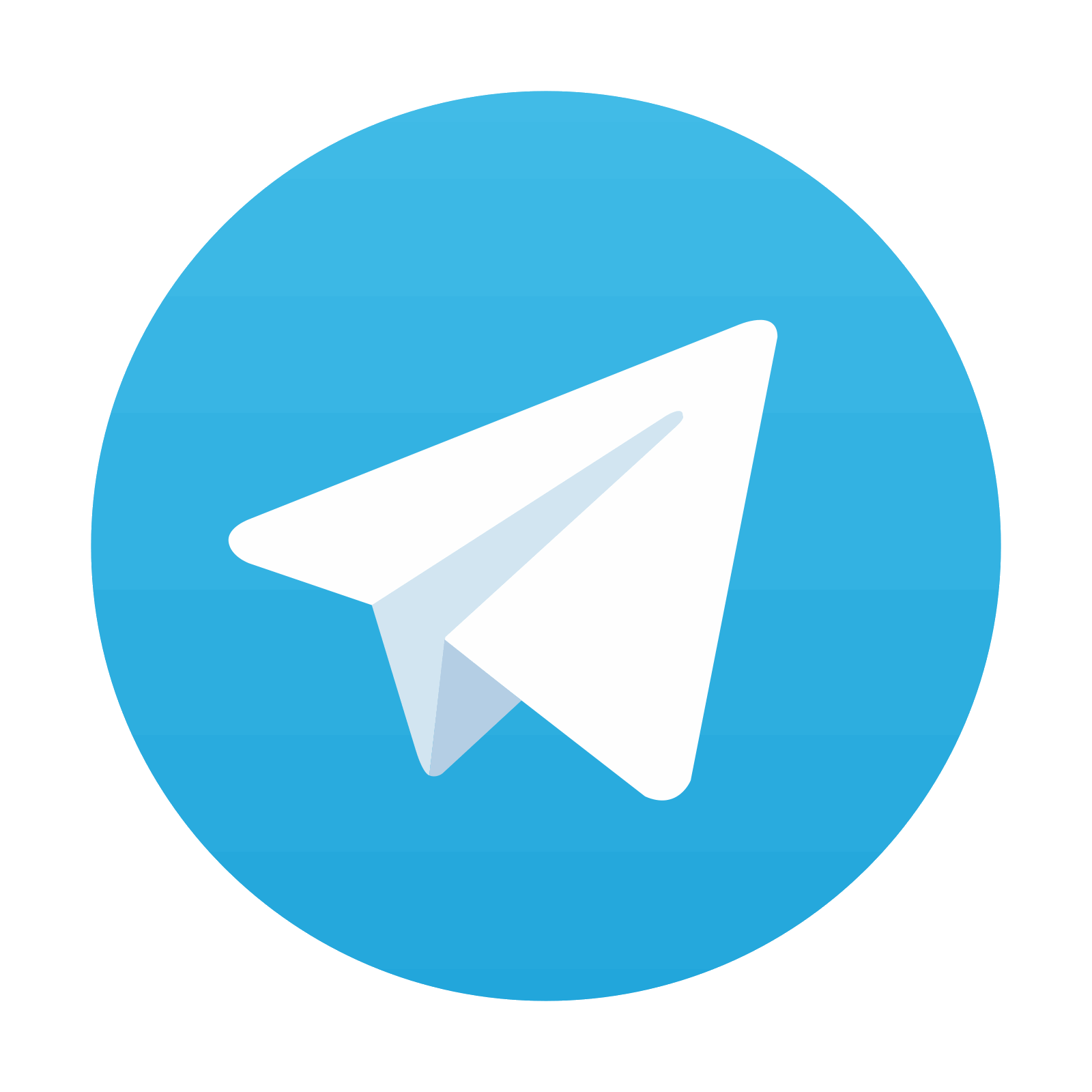
Stay updated, free articles. Join our Telegram channel
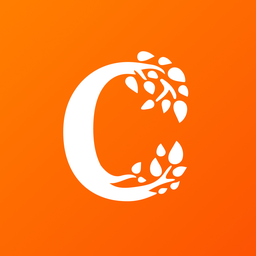
Full access? Get Clinical Tree
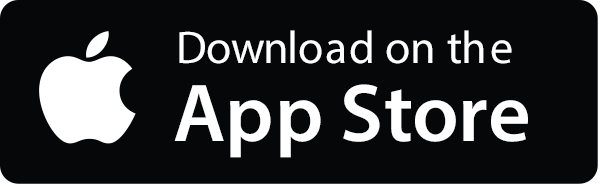
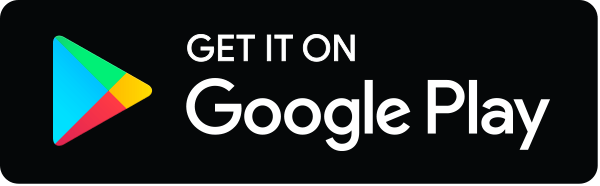