CHAPTER 15
The Visual System
Mammals are visual animals: The visual system conveys more information to the brain than any other afferent system. This information is processed within the brain so as to form a set of maps of the visual world. A relatively large proportion of brain tissue is devoted to vision. The visual system includes the eye and retina, the optic nerves, and the visual pathways within the brain, where multiple visual centers process information about different aspects (shape and form, color, motion) of visual stimuli.
THE EYE
The functions (and clinical correlations) of the cranial nerves (III, IV, VI) involved in moving the eyes have been discussed in Chapter 8, along with the gaze centers and pupillary reflexes. The vestibulo-ocular reflex is briefly explained in Chapter 17. This chapter discusses the form, function, and lesions of the optic system from the retina to the cerebrum.
Anatomy and Physiology
The optical components of the eye are the cornea, the pupillary opening of the iris, the lens, and the retina (Fig 15–1). Light passes through the first four components, the anterior chamber, and the vitreous to reach the retina; the point of fixation (direction of gaze) normally lines up with the fovea. The retina (which develops as a portion of the brain itself, and is considered by some neuroscientists to be a specialized part of the brain, located within the eye) transforms light into electrical impulses (Fig 15–2).
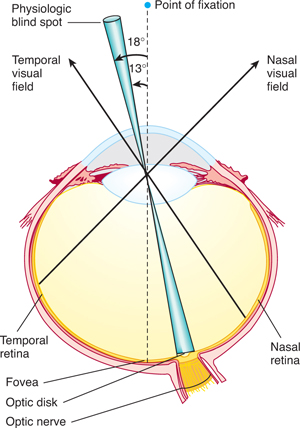
FIGURE 15–1 Horizontal section of the left eye; representation of the visual field at the level of the retina. The focus of the point of fixation is the fovea, the physiologic blind spot on the optic disk, the temporal (lateral) half of the visual field on the nasal side of the retina, and the nasal (medial) half of the visual field on the temporal side of the retina. (Reproduced, with permission, from Simon RP, Aminoff MJ, Greenberg DA: Clinical Neurology, 4th ed. Appleton & Lange, 1999.)
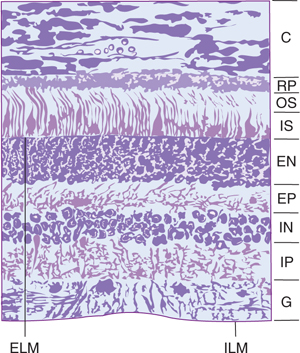
FIGURE 15–2 Section of the retina of a monkey. Light enters from the bottom and traverses the following layers: internal limiting membrane (ILM), ganglion cell layer (G), internal plexiform layer (IP), internal nuclear layer (IN) (bipolar neurons), external plexiform layer (EP), external nuclear layer (EN) (nuclei of rods and cones), external limiting membrane (ELM), inner segments of rods (IS) (narrow lines) and cones (triangular dark structures), outer segments of rods and cones (OS), retinal pigment epithelium (RP), and choroid (C). ×655.
The retina, organized into 10 layers, contains two types of photoreceptors (rods and cones) and four types of neurons (bipolar cells, ganglion cells, horizontal cells, and amacrine cells) (Figs 15–2 and 15–3). The photoreceptors (rods and cones, which are first-order neurons) synapse with bipolar cells (Fig 15–4). These, in turn, synapse with ganglion cells; the ganglion cells are third-order neurons whose axons converge to leave the eye within the optic nerve.
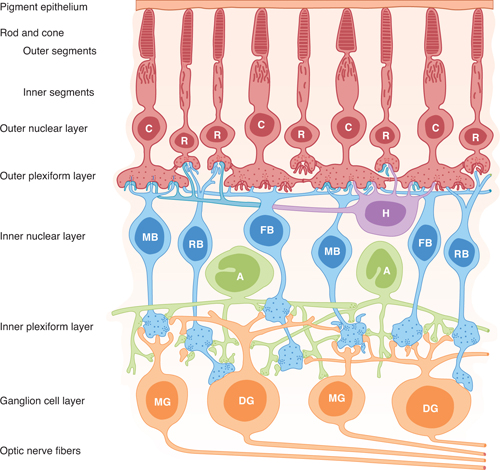
FIGURE 15–3 Neural components of the retina. C, cone; R, rod; MB, RB, FB, bipolar cells (of the midget, rod, and flat types, respectively); DG and MG, ganglion cells (of the diffuse and midget types, respectively); H, horizontal cells; A, amacrine cells. (Reproduced, with permission from Dowling JE, Boycott BB: Organization of the primate retina: Electron microscopy. Proc Roy Soc Lond Ser B [Biol] 1966;166:80.)
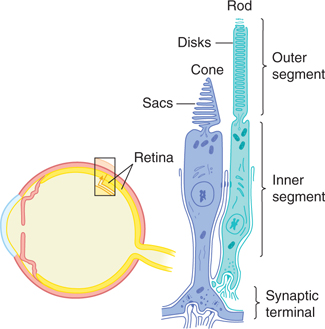
FIGURE 15–4 Schematic diagram of a rod and cone in the retina.
Within the outer plexiform layer of the retina, horizontal cells connect receptor cells with each other. Amacrine cells, within the inner plexiform layer, connect ganglion cells to one another (and in some cases also connect bipolar cells and ganglion cells).
Retinal Rods
Rods, which are more numerous than cones, are photoreceptors that are sensitive to low-intensity light and provide visual input when illumination is dim (eg, at twilight and at night). Cones are stimulated by relatively high-intensity light; they are responsible for sharp vision and color discrimination. Rods and cones each contain an outer segment, consisting of stacks of flattened disks of membrane that contain photosensitive pigments that react to light. In addition, they each have an inner segment, which contains the cell nucleus and mitochondria and forms synapses with the second-order bipolar cells. The transduction of light into neural signals occurs when photons are absorbed by photosensitive molecules (also called visual pigments) in the rods and cones.
The visual pigment within retinal rods is rhodopsin, a specialized membrane receptor that is linked to G-proteins. When light strikes the rhodopsin molecule, it is converted, first to metarhodopsin II and then to scotopsin and retinene1. This light-activated reaction activates a G-protein known as transducin, which breaks down cyclic guanosine monophosphate (GMP). Because cyclic GMP acts within the cytoplasm of the photoreceptors to keep sodium channels open, the lightinduced reduction in cyclic GMP leads to a closing of sodium channels, which causes a hyperpolarization (see Chapter 3). Thus, as a result of being struck by light, there is hyperpolarization within the retinal rods. This results in decreased release of synaptic transmitter onto bipolar cells, which alters their activity (Fig 15–5).
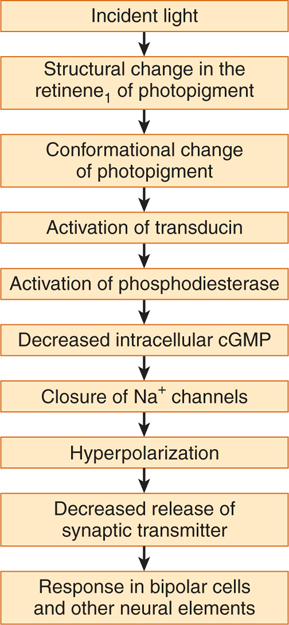
FIGURE 15–5 Probable sequence of events involved in phototransduction in rods and cones. cGMP, cyclic guanosine monophosphate. (Reproduced, with permission, from Ganong WF: Review of Medical Physiology, 22nd ed. McGraw-Hill, 2005.)
Retinal Cones
Cones within the retina also contain visual pigments, which respond maximally to light at wavelengths of 440, 535, and 565 nm (corresponding to the three primary colors: blue, green, and red). When cones are struck by light of the appropriate wavelength, a cascade of molecular events, similar to that in rods, activates a G-protein that closes sodium channels, resulting in hyperpolarization.
Bipolar, Amacrine, and Retinal Ganglion Cells
Transmission from photoreceptors (rods and cones, first-order sensory neurons) to bipolar cells (second-order sensory neurons) and then to retinal ganglion cells (third-order sensory neurons) is modified by horizontal cells and amacrine cells. Each bipolar cell receives input from 20 to 50 photoreceptor cells. The receptive field of the bipolar cell (ie, the area on the retina that influences the activity in the cell) is modified by horizontal cells. The horizontal cells form synapses on photoreceptors and nearby bipolar cells in a manner that “sharpens” the receptive field on each bipolar cell. As a result of this arrangement, bipolar cells do not merely respond to diffuse light; on the contrary, some bipolar cells convey information about small spots of light surrounded by darkness. (These cells have “on”-center receptive fields, whereas others convey information about small, dark spots surrounded by light [“off”-center receptive fields]).
Amacrine cells receive input from bipolar cells and synapse onto other bipolar cells near their sites of input to ganglion cells. Like horizontal cells, amacrine cells “sharpen” the responses of ganglion cells. Some ganglion cells respond most vigorously to a light spot surrounded by darkness, whereas others respond most actively to a dark spot surrounded by light.
The retinal area for central, fixated vision during good light is the macula (Fig 15–6). The inner layers of the retina in the macular area are pushed apart, forming the fovea centralis, a small, central pit composed of closely packed cones, where vision is sharpest and color discrimination is most acute.
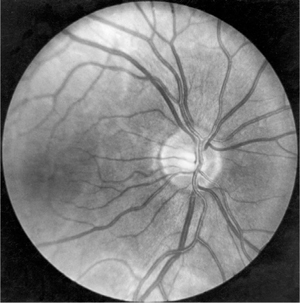
FIGURE 15–6 The normal fundus as seen through an ophthalmoscope. (Photo by Diane Beeston; Reproduced, with permission, from Riordan-Eva P, Whitcher JP: Vaughan & Asbury’s General Ophthalmology, 17th ed. McGraw-Hill, 2008.)
Retinal ganglion cells are specialized neurons that can be grouped into two classes that subserve different functions. Magnocellular ganglion cells have larger diameter axons (faster conduction velocities) and are sensitive to motion but not to color or details of form. Parvocellular ganglion cells have thinner axons (slower conduction velocities) and convey information about form and color. These two information streams converge on different layers of the lateral geniculate nucleus (see Visual Pathways section), an important central target.
Ganglion cell axons, within the retina, form the nerve fiber layer. The ganglion cell axons all leave the eye, forming the optic nerve, at a point 3 mm medial to the eye’s posterior pole. The point of exit is termed the optic disk and can be seen through the ophthalmoscope (see Fig 15–6). Because there are no rods or cones overlying the optic disk, it corresponds to a small blind spot in each eye.
A. Adaptation
If a person spends time in brightly lighted surroundings and then moves to a dimly lighted environment, the retinas slowly become more sensitive to light as the individual becomes accustomed to the dark. This decline in visual threshold, known as dark adaptation, is nearly maximal in about 20 minutes, although there is some further decline over longer periods. On the other hand, when one passes suddenly from a dim to a brightly lighted environment, the light seems intensely and even uncomfortably bright until the eyes adapt to the increased illumination and the visual threshold rises. This adaptation occurs over a period of about 5 minutes and is called light adaptation. The pupillary light reflex, which constricts the pupils, is normally a protective accompaniment to sudden increases in light intensity (see Chapter 8).
Light and dark adaptation depend, in part, on changes in the concentration of cyclic GMP in photoreceptors. In sustained illumination, there is a reduction in the concentration of calcium ions within the photoreceptor, which leads to increased guanylate cyclase activity and increased cyclic GMP levels. This, in turn, tends to keep sodium channels open so as to desensitize the photoreceptor.
B. Color Vision
The portion of the spectrum that stimulates the retina to produce sight ranges from 400 to 800 nm. Stimulation of the normal eye, either by this entire range of wavelengths or by mixtures from certain different parts of the range, produces the sensation of white light. Monochromatic radiation from one part of the spectrum is perceived as a specific color or hue. The Young-Helmholtz theory postulates that the retina contains three types of cones, each with a different photopigment maximally sensitive to one of the primary colors (red, blue, and green). The sensation of any given color is determined by the relative frequency of impulses from each type of cone. Parvocellular ganglion cells receive color-specific signals from the three types of cones and relay them to the brain via the optic nerve.
Each of the three photopigments has been identified. The amino acid sequences of all three are about 41% homologous with rhodopsin. The green-sensitive and red-sensitive pigments are very similar (about 40% homologous with each other) and are coded by the same chromosome. The bluesensitive pigment is only about 43% homologous with the other two and is coded by a different chromosome.
In normal color (trichromatic) vision, the human eye can perceive the three primary colors and can mix these in suitable portions to match white or any color of the spectrum. Color blindness can result from a weakness of one cone system or from dichromatic vision, in which only two cone systems are present. In the latter case, only one pair of primary colors is perceived; the two colors are complementary to each other. Most dichromats are red-green blind and confuse red, yellow, and green. Color blindness tests use special cards or colored pieces of yarn.
C. Accommodation
The lens is held in place by fibers between the lens capsule and the ciliary body (Figs 15–1 and 15–7). In the unaccommodated state, these elastic fibers are taut and keep the lens somewhat flattened. In the accommodated state, contraction of the circular ciliary muscle slackens the tension on the elastic fibers, and the lens, which has an intrinsic capacity to become rounder, assumes a more biconvex shape. The ciliary muscle is a smooth muscle that is innervated by the parasympathetic system (cranial nerve III; see Chapter 8); it can be paralyzed with atropine or similar drugs.
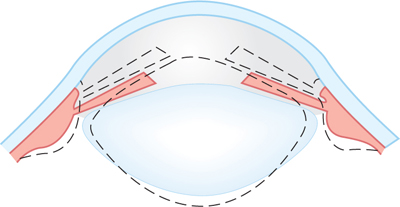
FIGURE 15–7 Accommodation. The solid lines represent the shape of the lens, iris, and ciliary body at rest, and the dotted lines represent the shape during accommodation. (Reproduced, with permission, from Ganong WF: Review of Medical Physiology, 22nd ed. McGraw-Hill, 2005.)
D. Refraction
When one views a distant object, the normal (emmetropic) eye is unaccommodated and the object is in focus. A normal eye readily focuses an image of a distant object on its retina, 24 mm behind the cornea; the focal length of the optics and the distance from cornea to retina are well matched, a state known as emmetropia (Fig 15–8). To bring closer objects into focus, the eye must increase its refractive power by accommodation. The ability of the lens to do so decreases with age as the lens loses its elasticity and hardens. The effect on vision usually becomes noticeable at approximately 40 years of age; by the 50s, accommodation is generally lost (presbyopia).
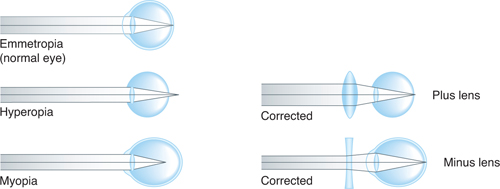
FIGURE 15–8 Emmetropia (normal eye) and hyperopia and myopia (common defects of the eye). In hyperopia, the eyeball is too short, and light rays come to a focus behind the retina. A biconvex lens corrects this by adding to the refractive power of the lens of the eye. In myopia, the eyeball is too long, and light rays focus in front of the retina. Placing a biconcave lens in front of the eye causes the light rays to diverge slightly before striking the eye, so that they are brought to a focus on the retina. (Reproduced, with permission, from Ganong WF: Review of Medical Physiology, 22nd ed. McGraw-Hill, 2005.)
Tests of Visual Function
In assessing visual acuity, distant vision is tested with Snellen or similar cards for persons with fairly normal vision. Finger counting and finger movement tests are used for those with subnormal vision, and light perception and projection for those with markedly subnormal vision. Near vision is tested with standard reading cards.
Perimetry is used to determine the visual fields (Fig 15–9). The field for each eye (monocular field) is plotted with a device or by the confrontation method to determine the presence of a scotoma or other field defect (see discussion of Clinical Correlations under Visual Pathways section). Normally, the visual fields overlap in an area of binocular vision (Fig 15–10).
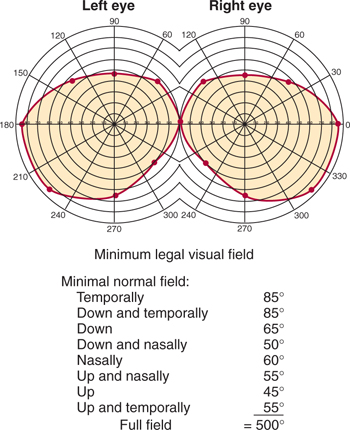
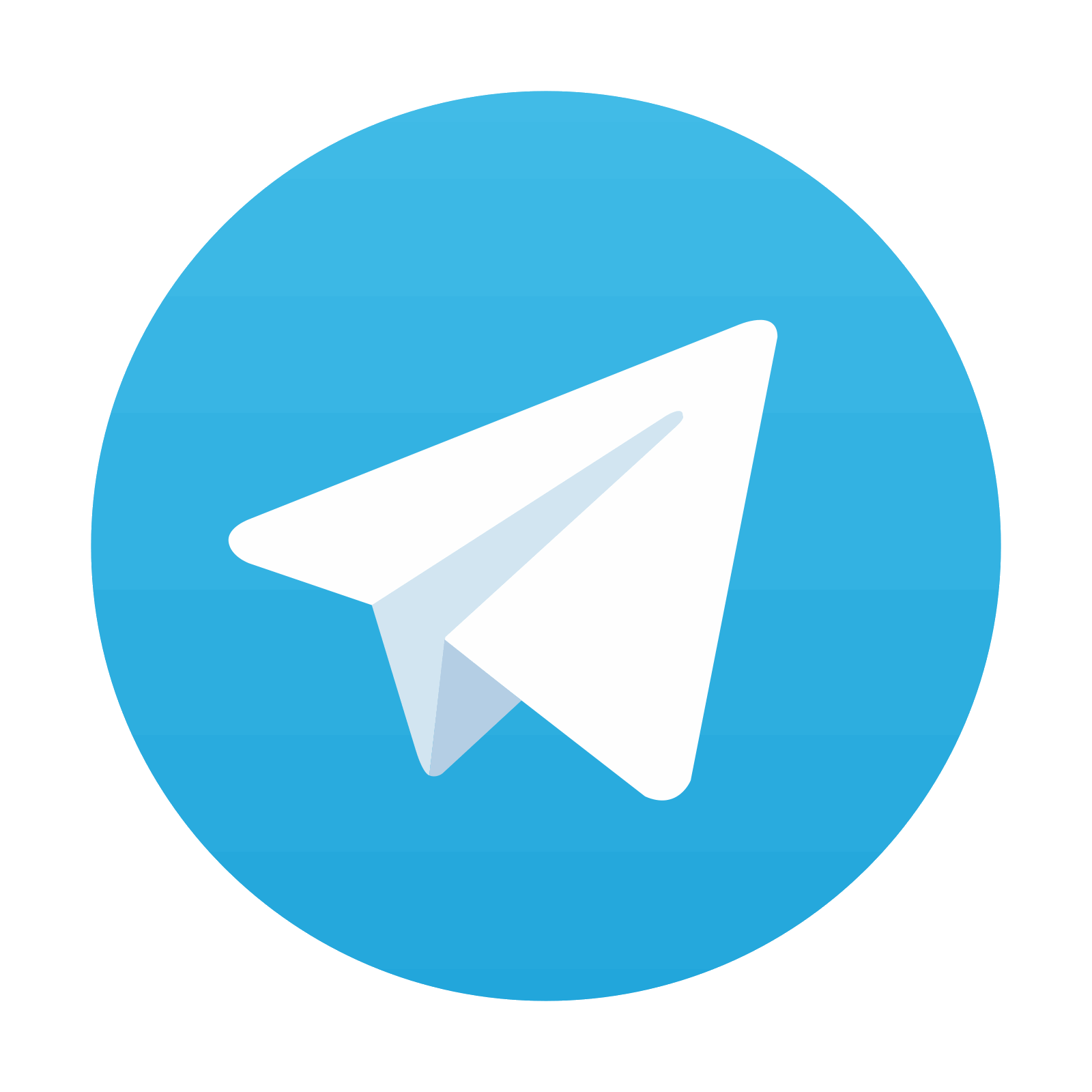
Stay updated, free articles. Join our Telegram channel
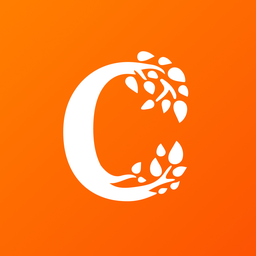
Full access? Get Clinical Tree
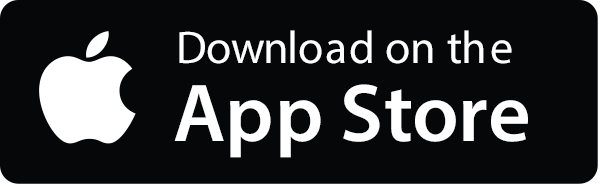
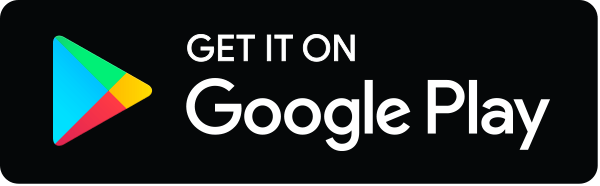