Transmitter Release
Transmitter Release Is Regulated by Depolarization of the Presynaptic Terminal
Release Is Triggered by Calcium Influx
The Relation Between Presynaptic Calcium Concentration and Release
Several Classes of Calcium Channels Mediate Transmitter Release
Transmitter Is Released in Quantal Units
Transmitter Is Stored and Released by Synaptic Vesicles
Synaptic Vesicles Discharge Transmitter by Exocytosis and Are Recycled by Endocytosis
Capacitance Measurements Provide Insight into the Kinetics of Exocytosis and Endocytosis
Exocytosis Involves the Formation of a Temporary Fusion Pore
Exocytosis of Synaptic Vesicles Relies on a Highly Conserved Protein Machinery
The Synapsins Are Important for Vesicle Restraint and Mobilization
SNARE Proteins Catalyze Fusion of Vesicles with the Plasma Membrane
Calcium Binding to Synaptotagmin Triggers Transmitter Release
The Fusion Machinery Is Embedded in a Conserved Protein Scaffold at the Active Zone
Modulation of Transmitter Release Underlies Synaptic Plasticity
Activity-Dependent Changes in Intracellular Free Calcium Can Produce Long-Lasting Changes in Release
Axo-axonic Synapses on Presynaptic Terminals Regulate Transmitter Release
SOME OF THE BRAIN’S MOST remarkable abilities, such as learning and memory, are thought to emerge from the elementary properties of chemical synapses, where presynaptic terminals release chemical transmitters that activate receptors in the membrane of the postsynaptic cell. In the last three chapters we saw how postsynaptic receptors control ion channels that generate the postsynaptic potential. Here we consider how electrical and biochemical events in the presynaptic terminal lead to the secretion of neurotransmitters. In the next chapter we examine the chemistry of the neurotransmitters themselves.
Transmitter Release Is Regulated by Depolarization of the Presynaptic Terminal
What are the signals at the presynaptic terminal that lead to the release of transmitter? Bernard Katz and Ricardo Miledi first demonstrated the importance of depolarization of the presynaptic membrane through the firing of a presynaptic action potential. For this purpose they used the giant synapse of the squid, a synapse large enough to permit insertion of electrodes into both pre- and postsynaptic structures. Two electrodes are inserted into the presynaptic terminal—one for stimulating and one for recording—and one electrode is inserted into the postsynaptic cell for recording the excitatory postsynaptic potential (EPSP), which provides an index of transmitter release (Figure 12–1A).
Figure 12-1 Transmitter release is triggered by changes in presynaptic membrane potential. (Adapted, with permission, from Katz and Miledi 1967a.)
A. Voltage recording electrodes are inserted in both the pre- and postsynaptic fibers of the giant synapse in the stellate ganglion of a squid. A current-passing electrode is also inserted presynaptically to elicit a presynaptic action potential.
B. Tetrodotoxin (TTX) is added to the solution bathing the cell to block the voltage-gated Na+ channels that underlie the action potential. The amplitudes of both the presynaptic action potential and the excitatory postsynaptic potential (EPSP) gradually decrease as more and more Na+ channels are blocked. After 7 min the presynaptic action potential can still produce a supra-threshold EPSP that triggers an action potential in the postsynaptic cell. After about 14 to 15 min the presynaptic spike gradually becomes smaller and produces smaller postsynaptic depolarizations. When the presynaptic spike is reduced to 40 mV or less, it fails to produce an EPSP. Thus the size of the presynaptic depolarization (here provided by the action potential) controls the magnitude of transmitter release.
C. An input–output curve for transmitter release is determined from the dependence of the amplitude of the EPSP on the amplitude of the presynaptic action potential. This relation is obtained by stimulating the presynaptic nerve during the onset of the blockade by TTX of the presynaptic Na+ channels, when there is a progressive reduction in the amplitude of the presynaptic action potential and postsynaptic depolarization. The upper plot demonstrates that (1) a 40 mV presynaptic action potential is required to produce a postsynaptic potential. Beyond this threshold there is a steep increase in amplitude of the EPSP in response to small increases in the amplitude of the presynaptic potential and (2) the relationship between the presynaptic spike and the EPSP is logarithmic, as shown in the lower plot. A 10 mV increase in the presynaptic spike produces a 10-fold increase in the EPSP.
When the presynaptic neuron is stimulated it fires an action potential, and after a brief delay an EPSP large enough to trigger an action potential is recorded in the postsynaptic cell. Katz and Miledi then asked how the presynaptic action potential triggers transmitter release. They found that as voltage-gated Na+ channels are blocked by application of tetrodotoxin, successive action potentials become progressively smaller. As the action potential is reduced in size, the EPSP decreases accordingly (Figure 12-1B). When the Na+ channel blockade becomes so profound as to reduce the amplitude of the presynaptic spike below 40 mV (positive to the resting potential), the EPSP disappears altogether. Thus the amount of transmitter release (as measured by the size of the postsynaptic depolarization) is a steep function of the amount of presynaptic depolarization (Figure 12-1C).
Katz and Miledi next investigated how presynaptic depolarization triggers transmitter release. The action potential is produced by an influx of Na+, and an efflux of K+ through voltage-gated channels. To determine whether Na+ influx or K+ efflux is required to trigger transmitter release, Katz and Miledi first blocked the Na+ channels with tetrodotoxin. They then asked whether direct depolarization of the presynaptic membrane, by current injection, would still trigger transmitter release. Indeed, depolarization of the presynaptic membrane beyond a threshold of about 40 mV positive to the resting potential elicits an EPSP in the postsynaptic cell. Beyond that threshold, progressively greater depolarization leads to progressively greater amounts of transmitter release. This result shows that during a normal action potential presynaptic Na+ influx is not necessary for release. Rather Na+ influx is important only insofar as it depolarizes the membrane enough for transmitter release to occur (Figure 12–2B).
Figure 12-2 Transmitter release is not directly triggered by the opening of presynaptic voltage-gated Na+ or K+ channels. (Adapted, with permission, from Katz and Miledi 1967a.)
A. Voltage recording electrodes are inserted in both the pre- and postsynaptic fibers of the giant synapse in the stellate ganglion of a squid. A current-passing electrode has also been inserted into the presynaptic cell.
B. Depolarizing the presynaptic terminal with direct current injection through a microelectrode can trigger transmitter release even after the voltage-gated Na+ channels are completely blocked by adding tetrodotoxin (TTX) to the cell-bathing solution. Three sets of traces represent (from bottom to top) the depolarizing current pulse injected into the presynaptic terminal (I), the resulting potential in the presynaptic terminal (Pre), and the EPSP generated by the release of transmitter onto the postsynaptic cell (Post). Progressively stronger current pulses in the presynaptic cell produce correspondingly greater depolarizations of the presynaptic terminal. The greater the presynaptic depolarization, the larger the EPSP. The presynaptic depolarizations are not maintained throughout the duration of the depolarizing current pulse because delayed activation of the voltage-gated K+ channels causes repolarization.
C. Transmitter release occurs even after the voltage-gated Na+ channels have been blocked with TTX and the voltage-gated K+ channels have been blocked with tetraethylammonium (TEA). In this experiment TEA was injected into the presynaptic terminal. The three sets of traces represent the same measurements as in part B. Because the presynaptic K+ channels are blocked, the presynaptic depolarization is maintained throughout the current pulse. The large sustained presynaptic depolarization produces large sustained EPSPs.
D. Blocking both the Na+, and K+ channels permits accurate control of presynaptic voltage and the determination of a complete input–output curve. Beyond a certain threshold (40 mV positive to the resting potential) there is a steep relationship between presynaptic depolarization and transmitter release, as measured from the size of the EPSP. Depolarizations greater than a certain level do not cause any additional release of transmitter. The initial presynaptic resting membrane potential was approximately -70 mV.
To examine the contribution of K+ efflux to transmitter release, Katz and Miledi blocked the voltage-gated K+ channels with tetraethylammonium at the same time they blocked the voltage-sensitive Na+ channels with tetrodotoxin. They then injected a depolarizing current into the presynaptic terminals and found that the EPSPs were of normal size, indicating that normal transmitter release occurred (Figure 12-2C). Thus neither Na+ nor K+ flux is required for transmitter release.
In the presence of tetraethylammonium the current pulse elicits a maintained presynaptic depolarization because the K+ current that normally repolarizes the presynaptic membrane is blocked. As a result, transmitter release is sustained throughout the current pulse as reflected in the prolonged depolarization of the postsynaptic cell. The sustained depolarization increased the accuracy of the measurements and permitted Katz and Miledi to determine a complete input–output curve relating presynaptic depolarization to transmitter release (Figure 12-2D). They confirmed the steep dependence of transmitter release on presynaptic depolarization. In the range of depolarization over which transmitter release increases (40–70 mV positive to the resting level) a 10 mV increase in depolarization produces a 10-fold increase in transmitter release. Depolarization of the presynaptic membrane above an upper limit produces no further increase in the postsynaptic potential.
Release Is Triggered by Calcium Influx
Katz and Miledi next turned their attention to Ca2+ ions. Earlier, Katz and José del Castillo had found that increasing the extracellular Ca2+ concentration enhanced transmitter release, whereas lowering the concentration reduced and ultimately blocked synaptic transmission. Because transmitter release is an intracellular process, these findings implied that Ca2+ must enter the cell to influence transmitter release.
Previous work on the squid giant axon membrane had identified a class of voltage-gated Ca2+ channels, the opening of which results in a large Ca2+ influx because of the large inward electrochemical driving force on Ca2+. The extracellular Ca2+ concentration, approximately 2 mM in vertebrates, is normally four orders of magnitude greater than the intracellular concentration, approximately 10-7 M at rest. However, because these Ca2+ channels are sparsely distributed along the axon they cannot, by themselves, provide enough current to produce a regenerative action potential.
Katz and Miledi found that the Ca2+ channels were much more abundant at the presynaptic terminal. There, in the presence of tetraethylammonium and tetrodotoxin, a depolarizing current pulse was sometimes able to trigger a regenerative depolarization that required extracellular calcium, a calcium spike. Katz and Miledi therefore proposed that Ca2+ serves dual functions. It is a carrier of depolarizing charge during the action potential (like Na+), and it is a special chemical signal—a second messenger—conveying information about changes in membrane potential to the intracellular machinery responsible for transmitter release. Calcium ions are able to serve as an efficient chemical signal because of their low resting concentration, approximately 105 fold lower than the resting concentration of Na+. As a result, the small amounts of ions that enter or leave a cell during an action potential can lead to large percentage changes in intracellular Ca2+ that can trigger various biochemical reactions. Proof of the importance of Ca2+ channels in release has come from more recent experiments showing that specific toxins that block Ca2+ channels also block release.
The properties of the voltage-gated Ca2+ channels at the squid presynaptic terminal were measured by Rodolfo Llinás and his colleagues. Using a voltage clamp, Llinás depolarized the terminal while blocking the voltage-gated Na+ channels with tetrodotoxin and the K+ channels with tetraethylammonium. He found that graded depolarizations activated a graded inward Ca2+ current, which in turn resulted in graded release of transmitter (Figure 12–3). The Ca2+ current is graded because the Ca2+ channels are voltage-dependent like the voltage-gated Na+, and K+ channels. Calcium ion channels in squid terminals differ from Na+ channels, however, in that they do not inactivate quickly but stay open as long as the presynaptic depolarization lasts.
Figure 12-3 Transmitter release is regulated by Ca2+ influx into the presynaptic terminals through voltage-gated Ca2+ channels. The voltage-sensitive Na+, and K+ channels in a squid giant synapse were blocked by tetrodotoxin and tetraethyl-ammonium. The membrane of the presynaptic terminal was voltage-clamped and membrane potential stepped to six different command levels of depolarization (bottom). The amplitude of the postsynaptic depolarization (top) varies with the size of the presynaptic inward Ca2+ current (middle) because the amount of transmitter release is a function of the concentration of Ca2+ in the presynaptic terminal. The notch in the postsynaptic potential trace is an artifact that results from turning off the presynaptic command potential. (Adapted, with permission, from Llinás and Heuser 1977.)
Calcium ion channels are concentrated in pre-synaptic terminals at active zones, the sites where neurotransmitter is released, exactly opposite the postsynaptic receptors (Figure 12–4). Calcium ions do not diffuse long distances from their site of entry because free Ca2+ ions are rapidly buffered by calcium-binding proteins. As a result, Ca2+ influx creates a sharp local rise in Ca2+ concentration at the active zones. This rise in Ca2+ in the presynaptic terminals can be visualized using Ca2+-sensitive fluorescent dyes (Figure 12-4B). One striking feature of transmitter release at all synapses is its steep and nonlinear dependence on Ca2+ influx; a twofold increase in Ca2+ influx can increase the amount of transmitter released by 16-fold. This relationship indicates that at some site, the calcium sensor, the cooperative binding of several Ca2+ ions is required to trigger release.
Figure 12-4 Calcium flowing into the presynaptic nerve terminal during synaptic transmission at the neuromuscular junction is concentrated at the active zone. Calcium channels in presynaptic terminals at the end-plate are concentrated opposite clusters of nicotinic acetylcholine (ACh) receptors on the postsynaptic muscle membrane. Two drawings show the frog neuromuscular junction.
A. The enlarged view shows the microanatomy of the neuromuscular junction with the presynaptic terminal peeled back. A fluorescent image shows the presynaptic Ca2+ channels (labeled with a Texas red-coupled marine snail toxin that binds to Ca2+ channels), and postsynaptic ACh receptors (labeled with fluorescently tagged α-bungarotoxin, which binds selectively to ACh receptors). The two images are normally superimposed but have been separated for clarity. The patterns of labeling with both probes are in almost precise register, indicating that the active zone of the presynaptic neuron is in almost perfect alignment with the postsynaptic membrane containing the high concentration of ACh receptors. (Reproduced, with permission, from Robitaille, Adler, and Charlton 1990.)
B. Calcium influx in presynaptic terminals is localized at active zones. Calcium can be visualized using calcium-sensitive fluorescent dyes. 1. A presynaptic terminal at a neuromuscular junction filled with the dye fura-2 under resting conditions is shown in the black and white image. The fluorescence intensity of the dye changes as it binds Ca2+. In the color image, color-coded fluorescence intensity changes show local hot-spots of intracellular Ca2+ in response to a single presynaptic action potential. Red indicates regions with a large increase in Ca2+blue indicates regions with little increase in Ca2+. Regular peaks of Ca2+ are seen along the terminal, corresponding to the localization of Ca2+ channels at the active zones. 2. The color image shows a high-magnification view of the peak increase in terminal Ca2+ levels. The corresponding black-and-white image shows fluorescence labeling of nicotinic ACh receptors in the postsynaptic membrane, illustrating the close spatial correspondence between areas of presynaptic Ca2+ influx and areas of postsynaptic receptors. The scale bar represents 2 µm. (Reproduced, with permission, from Wachman et al. 2004.)
The Relation Between Presynaptic Calcium Concentration and Release
How much Ca2+ is necessary to induce release of neurotransmitters? To address this question Bert Sakmann and Erwin Neher and their colleagues measured synaptic transmission in the calyx of Held, a large synapse in the mammalian brain stem that is part of the auditory pathway. This synapse is specialized for very rapid and reliable transmission to allow for precise localization of sound in the environment.
The calyx forms a cup-like presynaptic terminal that engulfs a postsynaptic cell body (Figure 12–5A). To ensure that every action potential results in reliable synaptic transmission, the calyx synapse includes almost a thousand active zones that function as independent synapses. In contrast, synaptic terminals of a typical neuron in the brain contain only a single active zone. Because the calyx terminal is large, it is possible to insert electrodes into both the pre- and postsynaptic structures, much as with the squid giant synapse, and directly measure the synaptic coupling between the two compartments. This paired recording allows a precise determination of the time course of activity in the presynaptic and postsynaptic cells (Figure 12-5B).
These recordings revealed a brief lag of 1 to 2 ms between the onset of the presynaptic action potential and the postsynaptic excitatory synaptic potential, which accounts for what Sherrington termed the synaptic delay. Because Ca2+ channels open more slowly than Na+ channels, Ca2+ does not begin to enter the presynaptic terminal until the membrane has begun to repolarize. Surprisingly, once Ca2+ enters the terminal, transmitter is rapidly released with a delay of only a few hundred microseconds. Thus the synaptic delay is largely attributable to the time required to open Ca2+ channels. The astonishing speed of Ca2+ action indicates that, prior to Ca2+ influx, the biochemical machinery underlying the release process must already exist in a primed and ready state.
A presynaptic action potential normally produces only a brief rise in presynaptic Ca2+ concentration because the Ca2+ channels open only for a short time. In addition, Ca2+ influx is localized at the active zone. These two properties contribute to a concentrated local pulse of Ca2+ that induces a burst of transmitter release (Figure 12-5B). As we shall see later in this chapter, the duration of the action potential regulates the amount of Ca2+ that flows into the terminal and thus the amount of transmitter release.
Figure 12-5 The precise relation between presynaptic Ca2+, and transmitter release at a central synapse has been measured. (Reproduced, with permission, from Meinrenken, Borst, and Sakmann 2003, and Sun et al. 2007.)
A. The large presynaptic terminal of the calyx of Held in the mammalian brain stem (yellow) engulfs a postsynaptic cell body (blue). Fluorescence image on left shows a calyx filled with a calcium-sensitive dye.
B. Time courses of the presynaptic action potential, presynaptic Ca2+ current, transmitter release rate, postsynaptic current through glutamate receptors, and the postsynaptic action potential. The dashed lines indicate the timing of the peak responses for the Ca2+ current, transmitter release, and postsynaptic action potential.
C. Transmitter release is steeply dependent on the Ca2+ concentration in the presynaptic terminal. The calyx was loaded with a caged Ca2+ compound that releases its bound Ca2+ in response to a flash of ultraviolet light, and with a Ca2+ sensitive dye that allows measuring how much Ca2+ is released. By controlling the intensity of light one can regulate the increase in Ca2+ in the presynaptic terminal. The plot, on a logarithmic scale, shows the relation between the rate of vesicle release and intracellular Ca2+ concentration. The circles depict measurements from individual experiments, and the blue line depicts a fit of the data by a model that assumes that release is triggered by a major Ca2+ sensor that binds five Ca2+ ions, resulting in a Ca2+ cooperativity of five. Due to the non-linear relationship between Ca2+, and release small increments in Ca2+ at concentrations of more than one µm cause massive increases in release.
D. The release of transmitter from a vesicle requires the binding of five Ca2+ ions to a calcium-sensing synaptic vesicle protein. In the figure Ca2+ binding is shown to trigger exocytosis by binding to five sensors present on a single vesicle. In reality, a single sensor binds several Ca2+ ions to trigger release.
To determine how much Ca2+ is needed to trigger release, the Neher and Sakmann groups introduced into the presynaptic terminal an inactive form of Ca2+ that was complexed within a light-sensitive chemical cage. They also loaded the terminals with a fluorescent dye that alters its fluorescence upon binding Ca2+, and so can be used to assay the intracellular Ca2+ concentration. By uncaging the Ca2+ ions with a flash of light they could trigger transmitter release through a uniform, known increase in Ca2+ concentration. These experiments revealed that a rise in Ca2+ concentration of less than 1 µM is sufficient to induce release of some transmitter, but approximately 10 to 30 µM Ca2+ is required to release the amount normally observed during an action potential. Here again the relationship between Ca2+ concentration and transmitter release is highly nonlinear, consistent with a model in which four or five Ca2+ ions must bind to the Ca2+ sensor to trigger release (Figure 12–5C,D).
Several Classes of Calcium Channels Mediate Transmitter Release
Calcium channels are found in all nerve cells and in many non-neuronal cells. In skeletal and cardiac muscle cells Ca2+ channels are important for excitation-contraction coupling; in endocrine cells they mediate release of hormones. Neurons contain five classes of voltage-gated Ca2+ channels: the L-type, P/Q-type, N-type, R-type, and T-type, each encoded by distinct genes or gene families. Each type has specific biophysical and pharmacological properties and physiological functions (Table 12–1).
Calcium channels are multimeric proteins whose distinct properties are determined by their pore-forming subunit, the α1-subunit. The α1-subunit is homologous to the α-subunit of the voltage-gated Na+ channel, comprised of four repeats of a domain with six membrane-spanning segments that includes the S4 voltage-sensor and pore-lining P region (see Figure 7-14). Calcium channels also have auxiliary subunits (termed α2, β, γ, and Δ) that modify the properties of the channel formed by the α1-subunit.
Four of the voltage-gated Ca2+ channels—the L-type, P/Q-type, N-type, and R-type—require fairly strong depolarization to be activated (voltages positive to -40 to -20 mV are required) and thus are often referred to as high-voltage-activated Ca2+ channels. In contrast, T-type channels open in response to small depolarizations around the threshold for generating an action potential (-60 to -40 mV) and are therefore called low-voltage-activated Ca2+ channels. Because they are activated by small changes in membrane potential, the T-type channels help control excitability at the resting potential and are an important source of the excitatory current that drives the rhythmic pacemaker activity of certain cells in both brain and heart.
In neurons the rapid release of conventional transmitters associated with fast synaptic transmission is mediated mainly by the P/Q-type and N-type Ca2+ channels because these are the channel types concentrated at the active zone. The localization of N-type Ca2+ channels at the frog neuromuscular junction has been visualized using a fluorescence-labeled snail toxin that binds selectively to these channels (see Figure 12-4A). The L-type channels are not found in the active zone and thus do not normally contribute to the fast release of conventional transmitters such as ACh and glutamate. However, Ca2+ influx through L-type channels is important for slower forms of release that do not occur at specialized active zones, such as the release of neuropeptides from neurons and of hormones from endocrine cells. As we shall see below, regulation of Ca2+ influx into presynaptic terminals controls the amount of transmitter release and hence the strength of synaptic transmission.
Voltage-gated Ca2+ channels are responsible for certain acquired and genetic diseases. A point mutation in the α1-subunit of P/Q-type channels underlies an inherited form of migraine. A mutation in the α1-subunit of L-type channels inhibits the voltage-dependent inactivation of these channels, and underlies Timothy syndrome, a pervasive developmental disorder involving both impaired cognitive function and a severe form of autism. Patients with Lambert-Eaton syndrome, an autoimmune disease associated with muscle weakness, make antibodies to the L-type channel α1-subunit that decrease total Ca2+ current.
Transmitter Is Released in Quantal Units
How does the influx of Ca2+ trigger release? Katz and his colleagues provided the key insight into this question by showing that transmitter is released in discrete amounts they called quanta. Each quantum of transmitter produces a postsynaptic potential of fixed size, called the quantal synaptic potential. The total postsynaptic potential is made up of a large number of quantal potentials. EPSPs seem smoothly graded in amplitude only because each quantal (or unit) potential is small relative to the total potential.
Katz and Fatt obtained the first clue as to the quantal nature of synaptic transmission in 1951 when they observed spontaneous postsynaptic potentials of approximately 0.5 mV in the nerve-muscle synapse of the frog. Like end-plate potentials evoked by nerve stimulation, these small depolarizing responses were largest at the site of nerve-muscle contact and decayed electrotonically with distance (see Figure 9-5). Small spontaneous potentials have since been observed in mammalian muscle and in central neurons. Because the postsynaptic potentials at vertebrate nerve-muscle synapses are called end-plate potentials, Fatt and Katz called these spontaneous potentials miniature end-plate potentials.
Several results convinced Fatt and Katz that the miniature end-plate potentials represented responses to the release of small amounts of ACh, the neurotransmitter used at the nerve-muscle synapse. The time course of the miniature end-plate potentials and the effects of various drugs on them are indistinguishable from the properties of the end-plate potential. Like the end-plate potentials, the miniature end-plate potentials are enhanced and prolonged by prostigmine, a drug that blocks hydrolysis of ACh by acetylcholinesterase. Conversely, they are reduced and finally abolished by agents that block the ACh receptor. The miniature end-plate potentials represent responses to small packets of transmitter that are spontaneously released from the presynaptic nerve terminal in the absence of an action potential. Their frequency can be increased by a small depolarization of the presynaptic terminal. They disappear if the presynaptic motor nerve degenerates and reappear when a new motor synapse is formed.
What could account for the small, fixed size of the miniature end-plate potential (around 0.5–1 mV)? Del Castillo and Katz first tested the possibility that each event represents a response to the opening of a single ACh receptor-channel. Small amounts of ACh applied to the frog muscle end-plate elicited depolarizing postsynaptic responses that were much smaller than the 0.5 mV response of a miniature end-plate potential. This finding made it clear that the miniature end-plate potential represents the opening of more than one ACh receptor-channel. In fact, Katz and Miledi were later able to estimate the voltage response to the elementary current through a single ACh receptor-channel as being only approximately 0.3 µV (see Chapter 9). Based on this estimate a miniature end-plate potential of 0.5 mV would represent the summation of the elementary currents of approximately 2,000 channels. Later work showed that a miniature end-plate potential is the response to the synchronous release of approximately 5,000 molecules of ACh.
What is the relationship of the large end-plate potential evoked by nerve stimulation and the small, spontaneous miniature end-plate responses? This question was addressed by del Castillo and Katz in a study of synaptic signaling at the nerve-muscle synapse bathed in a solution low in Ca2+. Under this condition the end-plate potential is reduced markedly, from the normal 70 mV to about 0.5 to 2.5 mV. Moreover, the amplitude of each successive end-plate potential now varies randomly from one stimulus to the next; often no response can be detected at all (termed failures). However, the minimum response above zero—the unit EPSP in response to a presynaptic action potential—is identical in amplitude (approximately 0.5 mV) and shape to the spontaneous miniature end-plate potentials. Importantly, the amplitude of each end-plate potential is an integral multiple of the unit potential (Figure 12–6).
Figure 12-6 Neurotransmitter is released in fixed increments. Each increment or quantum of transmitter produces a unit excitatory postsynaptic potential (EPSP) of fixed amplitude. The amplitude of the EPSP evoked by nerve stimulation is thus equal to the amplitude of the unit EPSP multiplied by the number of quanta of transmitter released.
A. Intracellular recordings from a muscle fiber at the end-plate show the change in postsynaptic potential when eight consecutive stimuli of the same size are applied to the motor nerve. To reduce transmitter release and to keep the end-plate potentials small, the tissue is bathed in a calcium-deficient (and magnesium-rich) solution. The postsynaptic responses to the nerve stimulus vary. Two presynaptic impulses elicit no EPSP (failures), two produce unit potentials, and the others produce EPSPs that are approximately two to four times the amplitude of the unit potential. Note that the spontaneous miniature end-plate potentials (S), which occur at random intervals in the traces, are the same size as the unit potential. (Adapted, with permission, from Liley 1956.)
B. After many end-plate potentials are recorded, the number of EPSPs corresponding to a given amplitude is plotted as a function of this amplitude in the histogram shown here. The distribution of responses falls into a number of peaks. The first peak, at 0 mV, represents failures. The first peak of responses, at 0.4 mV, represents the unit potential, the smallest elicited response. The unit response has the same amplitude as the spontaneous miniature end-plate potentials (inset), indicating that the unit response is caused by the release of a single quantum of transmitter. The other peaks in the histogram are integral multiples of the amplitude of the unit potential; that is, responses are composed of two, three, four, or more quantal events.
The number of responses under each peak divided by the total number of events in the entire histogram is the probability that a single presynaptic action potential triggers the release of the number of quanta that corresponds to the peak. For example, if there are 30 events in the peak corresponding to the release of two quanta out of a total of 100 events recorded, the probability that a presynaptic action potential releases exactly two quanta is 30/100 or 0.3. This probability follows a Poisson distribution (red curve). This theoretical distribution is composed of the sum of several Gaussian functions. The spread of the unit peak (standard deviation of the Gaussian function) reflects the fact that the amount of transmitter in a quantum, and hence the amplitude of the quantal postsynaptic response, varies randomly about a mean value. The successive Gaussian peaks widen progressively because the variability associated with each quantal event sums linearly with the number of quanta. The distribution of amplitudes of the spontaneous miniature potentials (inset) is fit by a Gaussian curve whose width is identical to that of the Gaussian curve for the unit synaptic responses. (Adapted, with permission, from Boyd and Martin 1956.)
Now del Castillo and Katz could ask: How does the rise of intracellular Ca2+ that accompanies each action potential affect the release of transmitter? They found that increasing the external Ca2+ concentration does not change the amplitude of the unit synaptic potential. However, the proportion of failures decreases and the incidence of higher-amplitude responses (composed of multiple quantal units) increases. These observations show that an increase in external Ca2+ concentration does not enhance the size of a quantum of transmitter (that is, the number of ACh molecules in each quantum) but rather acts to increase the average number of quanta that are released in response to a presynaptic action potential (Box 12–1). The greater the Ca2+ influx into the terminal, the larger the number of transmitter quanta released.
Thus three findings led del Castillo and Katz to conclude that transmitter is released in packets with a fixed amount of transmitter, a quantum: The amplitude of the end-plate potential varies in a stepwise manner at low levels of ACh release, the amplitude of each step increase is an integral multiple of the unit potential, and the unit potential has the same mean amplitude as that of the spontaneous miniature end-plate potentials.
In the absence of an action potential the rate of quantal release is low—only one quantum per second is released spontaneously at the end-plate. In the presence of a normal concentration of extracellular Ca2+ the firing of an action potential in the presynaptic terminal at the vertebrate nerve-muscle synapse releases approximately 150 quanta, each approximately 0.5 mV in amplitude, resulting in a large end-plate potential. Thus when Ca2+ enters the presynaptic terminal during an action potential, it dramatically increases the rate of quantal release by a factor of 150,000, triggering the synchronous release of about 150 quanta in about one millisecond.
Transmitter Is Stored and Released by Synaptic Vesicles
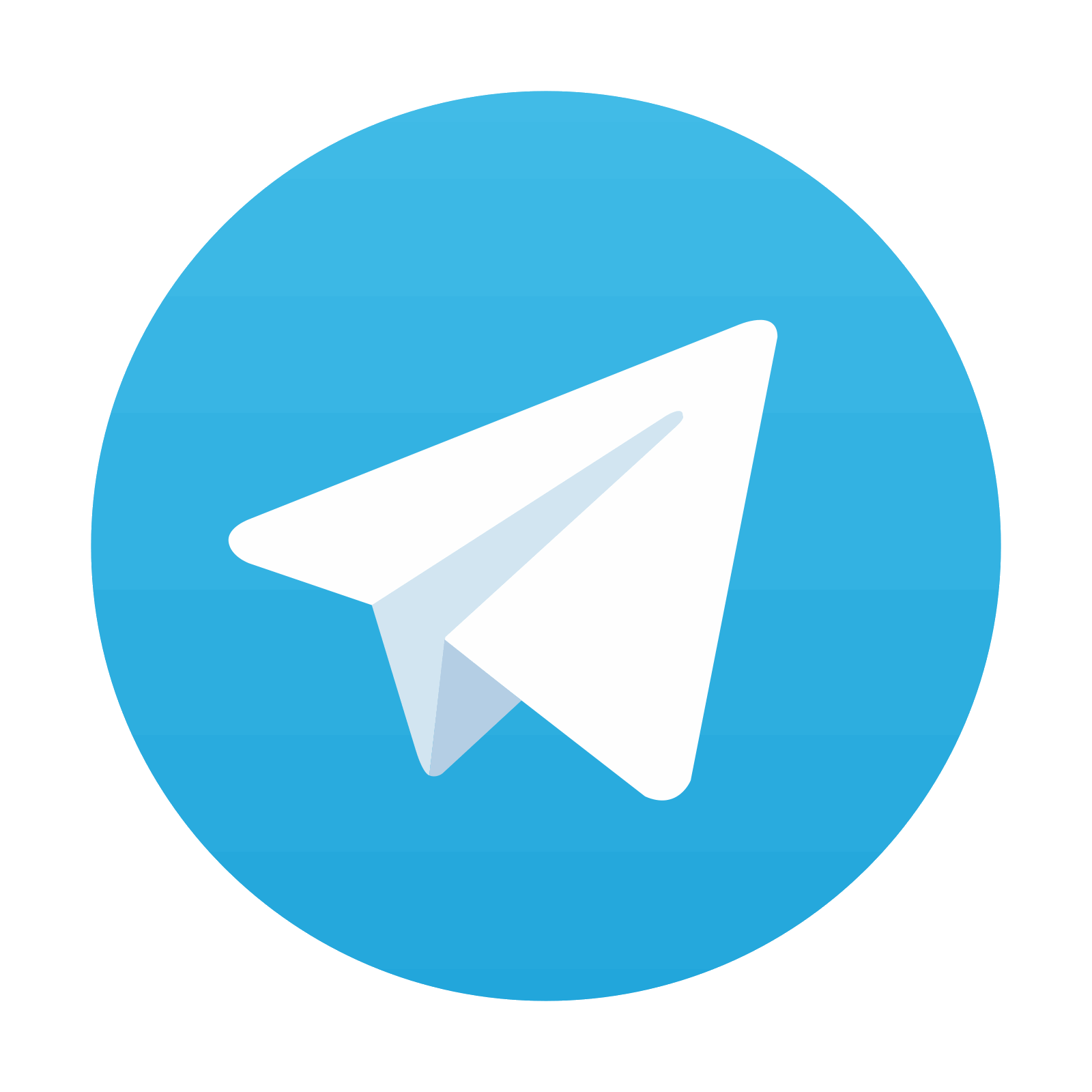
Stay updated, free articles. Join our Telegram channel
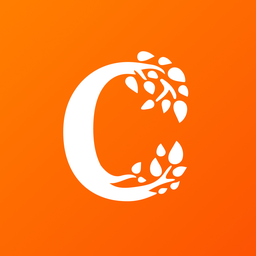
Full access? Get Clinical Tree
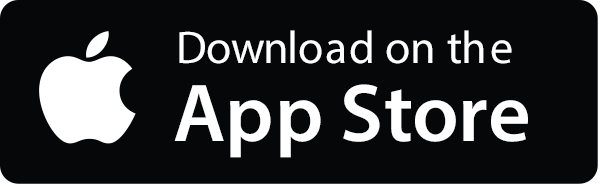
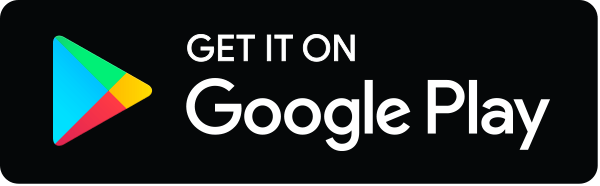